Scaffold-Based Tissue Engineering Strategies for Osteochondral Repair
- 1Department of Sports Medicine, Peking University Third Hospital, Beijing, China
- 2Institute of Sports Medicine of Peking University, Beijing, China
- 3Beijing National Laboratory for Molecular Sciences, State Key Laboratory of Polymer Physics and Chemistry, Institute of Chemistry, Chinese Academy of Sciences, Beijing, China
- 4University of Chinese Academy of Sciences, Beijing, China
Over centuries, several advances have been made in osteochondral (OC) tissue engineering to regenerate more biomimetic tissue. As an essential component of tissue engineering, scaffolds provide structural and functional support for cell growth and differentiation. Numerous scaffold types, such as porous, hydrogel, fibrous, microsphere, metal, composite and decellularized matrix, have been reported and evaluated for OC tissue regeneration in vitro and in vivo, with respective advantages and disadvantages. Unfortunately, due to the inherent complexity of organizational structure and the objective limitations of manufacturing technologies and biomaterials, we have not yet achieved stable and satisfactory effects of OC defects repair. In this review, we summarize the complicated gradients of natural OC tissue and then discuss various osteochondral tissue engineering strategies, focusing on scaffold design with abundant cell resources, material types, fabrication techniques and functional properties.
Introduction
The management and repair of osteochondral (OC) defects are still one of the most challenging clinical issues in orthopedics. Resulting from trauma, athletic injury or pathological factors, early localized osteochondral lesions can lead to general tissue deterioration, characterized clinically by severe pain and functional incapacitation of the affected joints (Hunter and Bierma-Zeinstra, 2019). As a common degenerative disease worldwide with high socioeconomic burdens, osteoarthritis (OA) is an adverse outcome of OC defects (Kwon et al., 2019). At the same time, OA can exacerbate the defects as a major cause. By 2030, approximately 67 millions people are expected to suffer from OA in the United States (Murphy and Helmick, 2012; Zhao et al., 2019). The upper articular cartilage possesses a stratified structure with no lymphatic or vascular components, lacking the capability of self-rehabilitation (Le et al., 2021). Moreover, different gradients of OC tissue have heterogeneous microstructures and biological properties (Ansari et al., 2019). So far, various clinical treatments have been available to alleviate symptoms and improve life quality to some extent, including microfracture technology, mosaicplasty, subchondral drilling, chondral shaving, abrasion arthroplasty, auto/allografts and joint replacement surgery (Redman et al., 2005; Gracitelli et al., 2016). Unfortunately, on account of the complex condition involving different layers of articular cartilage, cartilage-bone interface and subchondral bone, all these approaches have failed to achieve complete repair of OC defects and satisfactory reconstruction of joint functions. Then, the emergence of tissue engineering strategies has shown promise as a potential alternative for OC defect repair (Smith and Grande, 2015). This review aims to update the recent development in OC tissue engineering, focusing on biomaterial design and scaffold modification.
Native Osteochondral Tissue: Structure and Tissue Engineering
Articular Cartilage
As a tough and flexible connective tissue lack of lymphatics, blood vessels and nerves, articular cartilage can be further divided into the radial/deep zone, the transitional/middle zone and the superficial/tangential zone, consisting of embedded chondrocytes and extracellular matrix (ECM) (Hunziker et al., 2002; Sophia Fox et al., 2009) (Figure 1). As the singular cell type, chondrocytes are responsible for the synthesis, homeostasis and remodeling of ECM. Also, they can sense local environment by expressing integrins (Loeser, 2014). Each chondrocyte and the surrounded narrow pericellular matrix (PCM) is referred to as a chondron (Poole et al., 1987). With tensile strength and unique viscoelastic properties, articular cartilage facilitates load transmission to subchondral bone during compression and restores original appearance when the pressure is relieved, performed by the fibrillar collagen network and entrapped macromolecules such as collagen II and proteoglycans in the ECM (Carballo et al., 2017). In addition, articular cartilage provides a lubricated surface to reduce friction with the presence of lubricin and hyaluronic acid. In terms of nutritional supplies, on the one hand, joint movement and mechanical stimulation cause synovial fluid to flow over the cartilage surface. On the other hand, small molecules can penetrate from subchondral bone into articular cartilage through potential direct signaling pathways (Pan et al., 2009). Signals associated with cartilage injury or scaffold implantation can recruit and activate immune cells, followed by cellular polarization. The phenotypes of cells and their interactions can affect the local microenvironment. Pro-regenerative microenvironment can develop proper tissue resembling the original host tissue; however, unbalanced immune system can produce inflammation and fibrocartilage, causing functional impairment (Sadtler et al., 2016).
Calcified Cartilage
The calcified cartilage (CC) layer is defined as mineralized cartilage between articular cartilage layer and subchondral bone plate which passages from the so-called tidemark to the cement line (Ferguson et al., 2003; Lyons et al., 2006). The CC layer interlocked tightly with the upper articular cartilage and the lower subchondral bone plate in the manner of “ravine-engomphosis” and “comb-anchor”, respectively, (Wang et al., 2009) (Supplementary Figure S1). The undulated interface helps convert shear into compressive and tensile forces. Also, it can provide an integration to transfer mechanical load between flexible cartilage and stiff subchondral bone and maintain interfacial environment (Goldring and Goldring, 2016).
Subchondral Bone
Subchondral bone refers to the bony layer beneath the cement line, which can be anatomically divided into subchondral bone plate (SBP) and trabecular bone (STB) (Goldring and Goldring, 2010). SBP is impenetrable cortical lamellae, whereas STB is more porous and metabolically active with lower volume, density and stiffness. Osteocytes, the most widely distributed cell throughout bone tissue, are involved in bone metabolism and mechanical transduction through solid matrix directly or load-induced fluid flow indirectly (Knothe Tate et al., 2004; Furuya et al., 2018). Collagen I accounts for over 90% of bone matrix (Blair et al., 2017; Schlesinger et al., 2020). The environment in subchondral bone has an effect on the viscoelasticity and nutritional metabolism of articular cartilage (Burr and Gallant, 2012; Fell et al., 2019; Hu et al., 2021).
Gradients of the OC Tissue
Distinct gradients and properties during development and maturation, with regard to biochemistry, mechanics, architecture, electrics and metabolism, have been found in the OC tissue, which are not completely independent and act as the foundation for OC functional tissue engineering (Ansari et al., 2019; Li et al., 2021) (Figure 2).
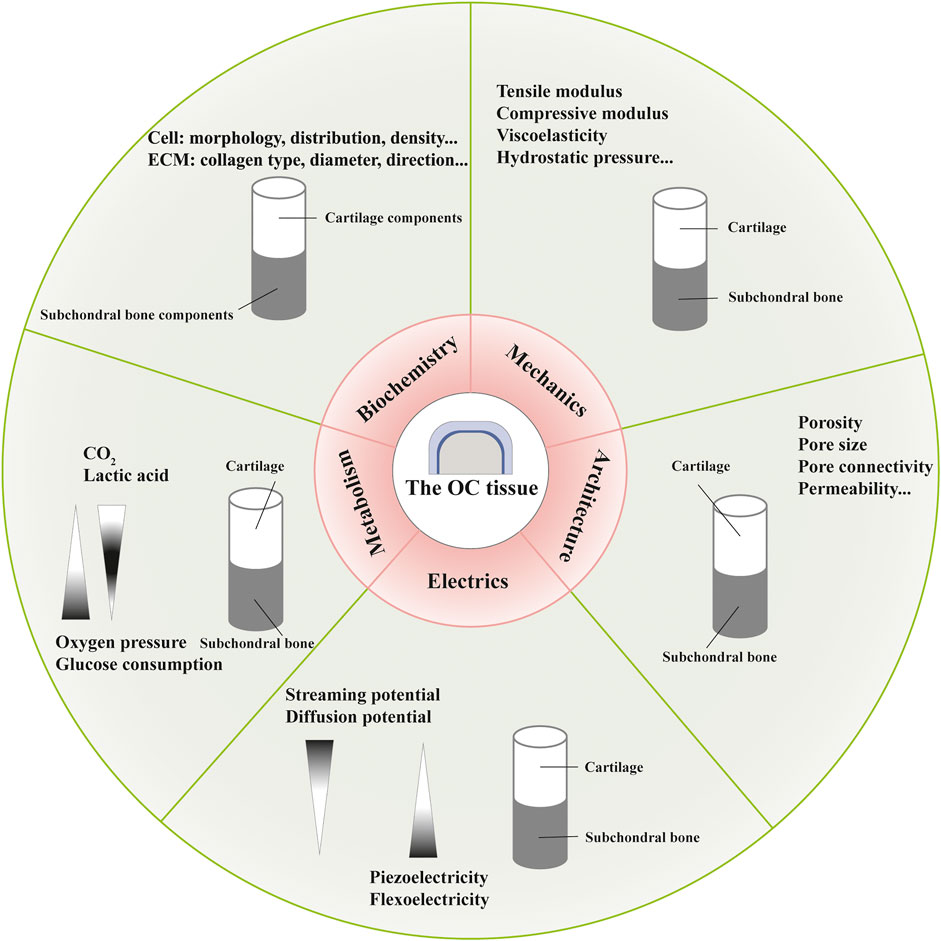
FIGURE 2. Different gradients in the osteochondral (OC) tissue with regard to biochemistry, mechanics, architecture, electrics and metabolism.
The OC tissue exhibits cellular and compositional transitions from articular cartilage layer to subchondral bone layer (Figure 1). Within the articular cartilage layer, gradients of cell morphology, distribution and surrounding ECM can be observed (Ren et al., 2016). In the superficial/tangential zone, the flattened chondrocytes with the maximum cell density and highly packed collagen fibers aligned parallel to the articular surface, endowing the cartilage with tensile properties. As an anatomic and functional bridge between the superficial and radial zones, the transitional/middle zone contains more rounded cells at low density and thicker collagen fibers which are organized obliquely, functioning as the first line of resistance to compressive forces. Chondrocytes in the radial/deep zone are ellipsoid or rounded, arranged in columns perpendicular to the joint surface. Also, collagen fibers in this zone are organized vertical to the surface. In the CC layer, we can see sparce chondrocytes with hypertrophic types which can produce collagen X. The underlying bone tissue comprises a variety of cells such as osteoblasts, osteoclasts, osteocytes, chondrocytes, endothelial cells and mesenchymal stem cells (MSCs) (Florencio-Silva et al., 2015). Moreover, hydroxyapatite increases gradually moving from articular cartilage to bone layer. The specific composition and content of different gradients should be considered when designing complicated layered constructs in OC tissue engineering. For instance, polymers with various water absorption capabilities can be used in combination to mimic moisture gradient. Different growth factors can be integrated to rehabilitate specific differentiation paths.
Essential constituents (collagen and proteoglycan, for instance) and mineralization degree lead to depth-dependent variations in mechanical properties including tensile modulus, compressive modulus, viscoelastic properties, and hydrostatic pressure (Responte et al., 2007; Chen et al., 2013; Armiento et al., 2018). Owing to the intrinsic material features of soft collagen fibers and rigid apatite crystals, and hierarchical arrangements more importantly, the bone layer develops diverse mechanical properties with regard to the loading direction as an anisotropic and viscoelastic material (Morgan et al., 2018). Accordingly, a collagen-apatite composite scaffold has been fabricated to restore bone-like hierarchical organization (Wingender et al., 2018). To repair OC tissue, metal alloys and ceramics have been used to mimic the strength and stiffness of subchondral layer, while polymers for the viscoelasticity of cartilage layer (Khorshidi and Karkhaneh, 2018). Fabrication techniques, such as electrospinning, can modify the mechanical properties of regenerated constructs by producing fibers with different diameters, components and structures. And bio-reactors can provided mechanical stimulation to mimic physiological conditions of OC tissue and control cell differentiation (Karkhaneh et al., 2014).
Architectural gradients refer to structural features such as porosity, pore size, pore connectivity and permeability. Articular cartilage has open and connected pores with a porosity of 60–85%, while the cortical bone and trabecular bone are 5–30% and 30–90% porous, respectively, (Mow et al., 1992; Di Luca et al., 2015). With permeability changing in a deformation, direction and location dependent manner, the articular cartilage inhibits fluid loss and promotes nutrition transmission (Maleki et al., 2020). And the permeability of bone tissue changes with the density of osteocytes and fabric parameters (Kreipke and Niebur, 2017). These architectural properties are closely related to cell migration and vascular in-growth in tissue regeneration.
At present, three theories exist about the electrical characteristics of the cartilage layer: streaming potential, diffusion potential and piezoelectricity (Schmidt-Rohlfing et al., 2002; More and Kapusetti, 2017; Farooqi et al., 2019). The bone tissue can also generate electricity under pressure possibly due to piezoelectricity of collagen and flexoelectricity of bone mineral (Vasquez-Sancho et al., 2018). Scaffolds produced by piezoelectric materials can provide electrical energy and trigger signaling pathways associated with cell morphology maintenance, gene expression and biological functions, associated with tissue repair (Jacob et al., 2018).
In terms of metabolism, different properties of tissues result from mediums of physical transport—synovial fluid and blood vessels, respectively. The metabolic gradients include oxygen pressure, glucose consumption and waste products, which are associated with chondrocyte phenotype, cellular functions and environmental homeostasis (Sheehy et al., 2012; Karner and Long, 2018; Sieber et al., 2020; Suzuki et al., 2020). Controlled oxygen releasing biomaterials, such as hyperoxide and fluorinated compounds, are potential candidate for oxygen gradient formation in OC tissue engineering (Camci-Unal et al., 2013). And well designed bio-reactors can provide nutrients and remove waste products (Hossain et al., 2014).
Undoubtedly, to design a suitable construct for the rehabilitation of detected OC tissue is based on comprehensive understanding of native structure and physiology. Necessarily, multi-layered scaffolds, which are synthesized respectively and assembled subsequently, can not form a smooth transition between different layers, probably resulting in unsatisfactory simulation of native tissue interface. Several methods, such as microfluidic system, centrifugation, core-shell and layer-by-layer deposition, have been used to mimic physical and chemical gradients of native osteochondral tissue (Cross et al., 2016; Ansari et al., 2019). Advances in bio-reactors and fabrication techniques pave the way for mimicking the complex microenvironment of OC tissue. And coinduction of mechanical and electrical gradients combined with stratified metabolic regulation of cells should be concerned in future studies.
Cell Sources in Osteochondral Tissue Engineering
In some OC tissue engineering strategies, scaffolds are preliminarily loaded with different cells to collectively promote tissue repair. Ideal cells for tissue engineering should have adequate sources and be able to maintain in vitro for manipulation and implantation safely. As shown in Table 1, two commonly proposed cell types for osteochondral repair are tissue-specific cells and progenitor cells, namely stem cells from different sources (Figure 3). Consistent with the host tissue, we usually use chondrocytes for hyaline cartilage repair and osteoblasts for subchondral bone regeneration. However, the use of differentiated cells suffers several limitations in the successive process of harvest, isolation, expansion, seeding, culture and finally implantation. For instance, chondrocytes are characterized by limited quantity in the native cartilage tissue, isolation difficulty and dedifferentiation capacity (Nukavarapu and Dorcemus, 2013). Since chondrocytes and osteoblasts both originate from bone marrow stem cells, MSCs have received widespread attention for their prominent advantages such as rapid proliferation and multipotency (Kagami et al., 2011; Mahmoudifar and Doran, 2013). Also, the secretory and immunomodulatory functions are closely related with cartilage regeneration. Various methods have been proposed for spatial and temporal control of differentiation toward the osteogenic and chondrogenic lineage including matrix properties and external factors, remaining to further explore and perfect (Seong et al., 2010; Mendes et al., 2018). Additionally, cell-free strategies have been pursued to overcome the aforementioned limitations (Maia et al., 2018). In combination with microfracture technology, biocompatible and biodegradable scaffolds without cells are implanted to promote cell recruitment and differentiation within the osteochondral defect area. Moreover, alternative approaches participated by MSC-derived exosomes or extracellular microvesicles have been developed in tissue repair and regeneration (Kim et al., 2020).
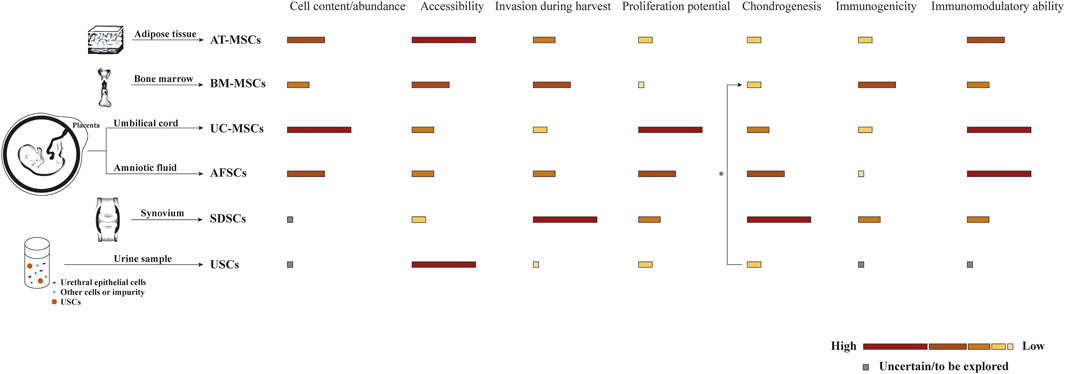
FIGURE 3. Comparison of stem cells from different sources commonly used in scaffold-based osteochondral (OC) tissue engineering strategies. Biological characteristics are shown as different color blocks from high to low, inspired by Zha et al. (2021). * USCs are reported to promote the chondrogenesis of BM-MSCs by paracrine action in the co-culture system (Gao et al., 2016).
Scaffold Fabrication Techniques
Scaffolds are one of the fundamental elements of tissue engineering approaches to osteochondral repair. For the quality of tissue regeneration, scaffolds are expected to have the following characteristics: porous structure for cell survival and material transport, suitable surface for cell adhesion, proliferation and differentiation, mechanical properties matching the surrounding tissue, biocompatibility with limited immunoreaction and bioabsorbability with a controllable degradation rate (Hutmacher, 2000).
To improve scalability, sustainability and spatial control, various methods for scaffold fabrication in osteochondral tissue engineering have been proposed, including lyophilization, freeze casting, gas foaming, sol-gel process, solvent casting, compression molding, particulate leaching and phase separation process et al. (Hutmacher, 2000; Cheng et al., 2019) (Table 2). Seo JP et al. utilized the freeze-drying technology to prepare bilayer gelatin/β-tricalcium phosphate (GT) sponges (Seo et al., 2013). In their study, PRP/BMP-2/GT scaffolds showed more cartilage-like tissue with no remaining implant materials and no evidence of infection, adhesions or synoyial proliferation. To establish various bioactive scaffolds, the use of freeze casting has drawn much concern in recent years. In a previous study, Abarrategi A et al. performed both in vitro and in vivo assays of cell survival and bone formation based on rhBMP-2/multiwall carbon nanotubes (MWCNT)/chitosan (CHI) scaffolds in conjunction with freeze casting (Abarrategi et al., 2008). The technology has high application value and development potential in forming structures in accordance with natural tissue (Shao et al., 2020). Reyes R et al. analyzed the effects of repair induced by TGF-β1/BMP-2 loaded segmented polyurethane/polylactic-co-glycolic (SPU/PLGA) scaffolds in osteochondral lesions (Reyes et al., 2014). The PLGA porous structure was produced by gas foaming in acidic aqueous solution. Algul D et al. manufactured multilayered β-TCP/chitosan-alginate polyelectrolyte complex (CA/PEC) scaffolds to mimic the structural gradients of native osteochondral tissue (Algul et al., 2015). The chitosan and alginate solutions were mixed and stirred to prepare a gel. The chitosan/alginate gel was treated through a series of steps for scaffold fabrication such as lyophilization, cross-linking and elution. Wu et al. evaluated the efficacy of bilayered silk scaffolds loaded with TGF-β3/BMP-2 for osteochondral defect repair in rabbits aided by the solvent casting/particulate leaching technology (Wu et al., 2021). The lyophilized silk powder was first mixed with hexafluoroisopropanol (HFIP) solution with or without sucrose particles in a silicone mold. Then, 10% silk-HFIP solution was poured onto the mold and kept for crosslink. The demolded scaffolds were treated with methanol (100% w/v) and running water to remove the HFIP and particles. After the process above, the top and bottom layers of scaffolds served as the cartilage and subchondral bone layers, respectively. Moreover, Sil-MA (methacrylated silk fibroin) hydrogels for marginal sealing were prepared by photo curing. This new approach indicated the effect of the marginal sealant on the integration of the cartilage layer and rapid cartilage formation. Duan et al. investigated the effects of pore size on osteochondral repair in vivo using a rabbit model (Duan et al., 2014). The bilayered poly(lactide-co-glycolide) (PLGA) porous scaffolds for research were fabricated by a compression molding/particulate leaching method. The PLGA solution and sodium chloride (NaCl) particulates as porogen were mixed and pressed into the pre-designed mold. After releasing the mold, cylindrical structure was obtained. Then, the mixture constructs were cut into appropriate sizes and leached by water. Five types of integrated scaffolds with identical porosity and different pore sizes were processed finally. The assessment in this study reminded us to take pore sizes into consideration during scaffold design for tissue engineering. Da et al. prepared the compact layer between the chondral and bony layers from PLGA/β-TCP by the phase separation process (Da et al., 2013). The homogeneous PLGA/β-TCP mixture was compactly extruded and solidified line by line above the subchondral bone layer. Through in vitro and in vivo tests, the compact layer-containing biphasic scaffolds showed better biomechanical properties and tissue repair results.
With the development of manufacturing, an advanced technique named electrospinning has been applied to produce functional scaffolds with spatially complex physical and chemical properties in osteochondral tissue engineering (Figure 4). To reduce the inflammatory immune responses, natural polymers such as collagen and silk fibroin have been employed as scaffold materials. Liu et al. fabricated a nanofiber yarn-collagen type I/hyaluronate hybrid (Yarn-CH)/TCP biphasic scaffold by dynamic liquid electrospinning, which showed an almost smooth articulating surface and good integrity of the host-implant interface (Liu S et al., 2015). Composite electrospun matrix derived from 70S bioactive glass and silk fibroin was obtained and evaluated as potential candidate for osteochondral defect repair by M JC et al. (M et al., 2017). The biphasic constructs showed the ability to synergistically support the chondrogenic and osteogenic growth. Also, certain synthetic polymers have been used as bioassimilable materials, including PCL, PLA and PLGA in particular (Liu W et al., 2015). The nanofibers fabricated by electrospinning technique can present a high surface area for cell growth and cellular differentiation (Aguirre-Chagala et al., 2017). Continuously graded insulin/PCL/β-GP scaffolds, fabricated via the application of the twin-screw extrusion/electrospinning method, have implemented the selective differentiation of h-ADSCs into chondrocytes and mineralized tissue hierarchically (Erisken et al., 2011). In the study of Baumgartner W et al., electrospun meshes of PLGA/amorphous calcium phosphate (aCaP) seeded with h-ADSCs were cultured in DMEM to explore the effects of aCaP and shear forces on osteogenic, chondrogenic, adipogenic and angiogenic stimulation (Baumgartner et al., 2019). To improve the reparative potential of irregular osteochondral defects, the cell/nanofibers composite electrospun scaffolds with a slurry-like texture have been produced. Kim HS et al. prepared the cartilage-dECM-decorated PCL electrospun scaffolds which were surface-modified with poly(glycidyl methacrylate) (PGMA@NF) via surface-initiated atom transfer radical polymerization (SI-ATRP) method (Kim et al., 2021). The in vivo study in a rat model indicated significantly improved cartilage and bone regeneration of osteochondral defects. To provide molecular cues and improve the spatiotemporal control of cell distribution and differentiation, Liu YY et al. reported a functional gradient scaffold consisting of gelatin/sodium alginate (SA) struts and electrospun nanofibers incorporated by gentamycin sulfate (GS) and desferoxamine (DFO) (Liu et al., 2016). Fabricated via a system which combined 3D biological printing and electrospinning process, the scaffold showed excellent mechanical stability. Loaded with different biomolecules, the 3D composite osteochondral scaffolds can achieve spatiotemporal release according to various requirements.
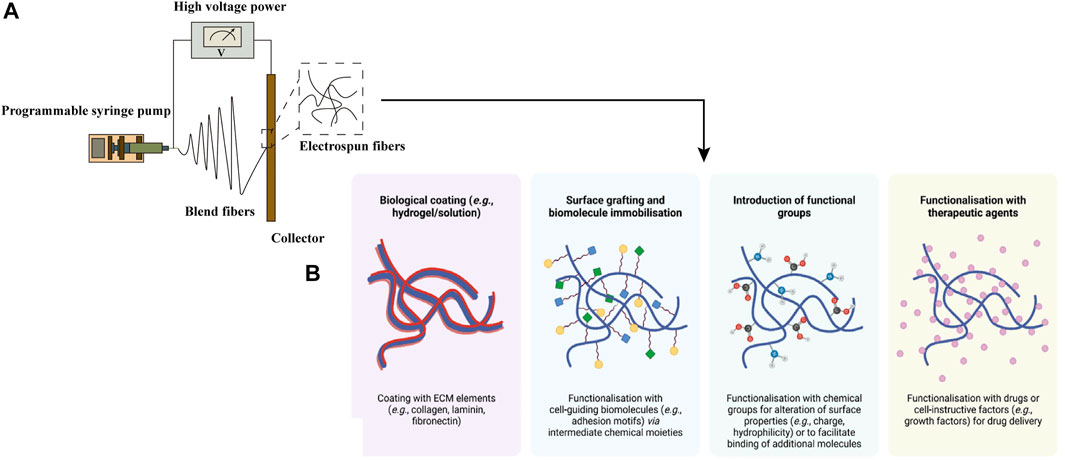
FIGURE 4. Electrospun fibers, obtained by basic electrospinning setup (A), can be modified by physical or chemical techniques. Post-fabrication surface modifications of electrospun fibers (B) were adapted with permission from ref (Gonçalves et al., 2021). Copyright 2021 by MDPI Inc.
Osteochondral scaffold manufacturing that can produce tunable structure in terms of architectural, biomechanical and biochemical properties is an ideal candidate for tissue engineering. Additive manufacturing (AM), an electrohydrodynamic technique, is gradually coming into focus. Characterized by layered sedimentation and computer-aided design/manufacturing (CAD/CAM) technology, AM methods include fused deposition modeling (FDM), selective laser sintering (SLS) and inkjet 3D printing et al. (Gonçalves et al., 2021). Ding C et al. designed a CAD/CAM-fabricated scaffold from polylactic acid-coated polyglycolic acid (PGA/PLA) and poly-ε-caprolactone/hydroxyapatite (PCL/HA) for goat femoral head regeneration (Ding et al., 2013). The PGA/PLA and PCL/HA scaffolds were respectively fabricated by 3D printing and FDM with aid of CAD/CAM technology. The strategy in this study provided a promising approach for tissue specific regeneration with cartilage tissue, immature calcified tissue, transitional trabecular bone and hypertrophic chondrocytes. Significantly, these methods can integrate bioactive molecules or drugs into scaffolds derived from various biomaterials. Tamjid E et al. prepared PCL composite scaffolds containing tetracycline hydrochloride (TCH) by 3D printing and tested the performance of biocompatibility, drug release kinetics and antibacterial activity (Tamjid et al., 2020). This study has paved the way for realizing sustainable release of loaded medicine. In another instance, an air-extrusion 3D printing technique was utilized to fabricate a scaffold consisting of poly(N-acryloyl 2-glycine)-methacrylated gelatin (PACG-GelMA)-Mn2+ for cartilage layer and PACG-GelMA-bioactive glass (BG) for bone layer (Gao et al., 2019). In vitro experiments indicated increased gene expression of both chondrogenic- and osteogenic-related differentiation. Also, the biohybrid scaffold promoted osteochondral tissue regeneration after implantation in a rat model. With excellent elasticity, hybrid scaffolds can support various deformation. In some cases, osteochondral defects are closely associated with mitochondrial dysfunction, metabolic reconfiguration and increased heterochromatin, which provides a potential avenue to design functional scaffolds for tissue regeneration (Varela-Eirin et al., 2018; Coryell et al., 2021). Chen P et al. examined the therapeutic potential of MSC-derived exosomes in 3D printed ECM/GelMA scaffolds (Chen et al., 2019). In vitro studies demonstrated increased chondrocyte migration by the scaffolds, which has been reported as a major element in the repair process of osteochondral defects (Chen et al., 2015; Zhang et al., 2018). The healing capacity was then assessed in a rabbit model. After implantation, the ECM/GelMA/exosome scaffold was shown to restore the damaged chondrocyte mitochondria by providing critical proteins and stimulate M2 macrophage polarization in the synovium. Advances in manufacturing have allowed the development of in situ 3D printing technology for osteochondral tissue repair, thereby improving the surgical procedure and the graft accuracy (Ma et al., 2020). However, mass industrial implementation and clinical applications are limited by several limitations: narrow range of suitable materials, time-consuming layer-by-layer processing and high costs, et al. Further exploration is needed to accelerate the clinical translation and fill the gap in osteochondral defect treatment.
Different Types of Scaffolds in Osteochondral Tissue Engineering
As a vital component of tissue engineering and regenerative medicine, scaffolds play an increasingly important role in the reconstruction and maintenance of tissue functions. Under the regulation of essential parameters, such as growth factors and functional particles, they can provide a platform for cell survival, proliferation and differentiation, thereby raising the possibility of tissue repair. Today, advances in biomaterials and fabrication techniques provide new opportunities for the development of biomimetic and sophisticated scaffolds, which can be further divided into monophasic, biphasic and multiphasic constructs. Apparently, multiphasic scaffolds have several advantages in bionic performance over the monophasic ones. In the following sections, we focus on different types of biphasic and multiphasic scaffolds for OC tissue engineering on the basis of biomaterial design (Supplementary Table S1).
Porous Scaffolds
There are various forms of porous scaffolds, including foam, sponge, mesh, microfibers and nanofibers, etc. The porous structure can function as an important support for cell aggregation and infiltration to guide further differentiation towards diverse lineages. Moreover, the interconnected pore networks with appropriate pore size and porosity are the forming basis of extracellular matrix simulating the native OC tissue. The resulting microenvironment can promote nutrient supply and stimulate effective cell-cell and cell-matrix communications. More significantly, the macro- and micro-pores of scaffolds are crucial channels for blood vessel and nerve growth. Considerable efforts have been made to optimize the structures of porous scaffolds to serve specific functions through tissue regeneration. As mentioned in the previous section, Duan P et al. first assessed the effects of pore size on the efficacy of in vivo osteochondral tissue repair (Figures 5A,B). Until now, researchers have not yet reached a consensus on the ideal pore morphology. In addition to pore size and porosity, more descriptive parameters (e.g., tortuosity and surface area to volume ratio, et al.) have been supplemented to characterize and evaluate scaffolds.
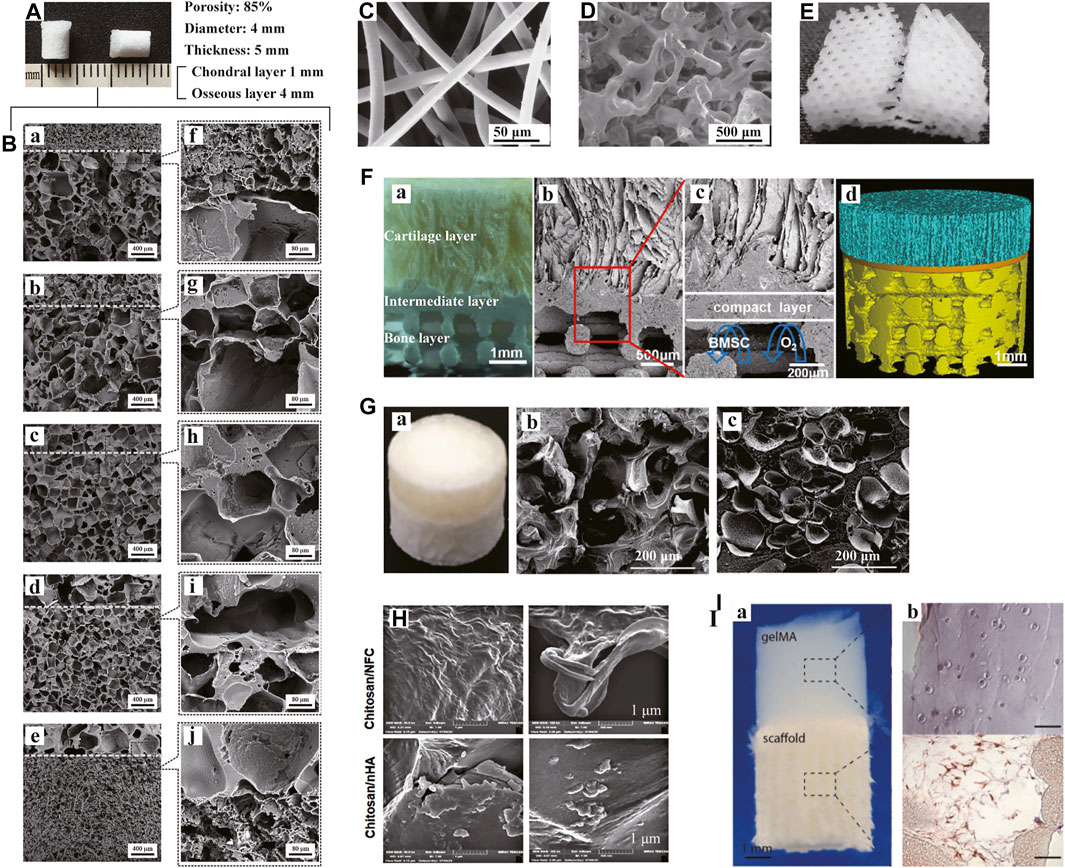
FIGURE 5. Porous and hydrogel scaffolds. Bilayered PLGA porous scaffolds (A) with different pore sizes shown in scanning electron microscopy (SEM) images (B: a-e). The boundaries between the layers were magnified (B: f-j). Bilayered porous scaffold consisting of non-woven PGA meshes (C) and PLGA/PEG foams (D). PCL porous scaffold with a 0/60/120° lay-down pattern (E). Muti-layered scaffolds with porous 3D printing PLGA/TCP bone layer (F) were shown in digital pictures (F: a), SEM images (F: b-c) and micro-CT reconstructive images (F: d), respectively. Bilayered CAN-PAC hydrogel (G: a) and the SEM images of upper (G: b) and lower layers (G: c). SEM images of the upper and lower layers of chitosan hydrogel scaffold (H). Tri-phasic scaffolds made of gelMA hydrogel and PCL/HA (I: a). CD31 immunohistochemical analysis showed the presence of HUVECs in the PCL-PCL/HA phase (I: b). (A, B) Adapted with permission from ref (Duan et al., 2014). Copyright 2013 by WILEY PERIODICALS Inc. (C, D) Adapted with permission from ref (Schaefer et al., 2000). Copyright 2000 by Elsevier Science Ltd. (E) Adapted with permission from ref (Cao et al., 2003). Copyright 2003 by Mary Ann Liebert Inc. (F) Adapted with permission from ref (Jia et al., 2018). Copyright 2018 by American Chemical Society. (G) Adapted with permission from ref (Liao et al., 2017). Copyright 2017 by Nature Publishing Group. (H) Adapted with permission from ref (Dehghani Nazhvani et al., 2021). Copyright 2021 by Elsevier Inc. (I) Adapted with permission from ref (Pirosa et al., 2021). Copyright 2021 by Elsevier Ltd.
Ideal porous scaffolds for clinical applications in osteochondral tissue engineering should meet both structural and functional requirements. Different layers of the scaffolds can mimic the natural gradients of the OC tissue. After implantation, the scaffolds can stimulate tissue regeneration and integration with the surrounding cartilage and subchondral bone. Natural and synthetic polymers are commonly employed as biomaterials. In an earlier in vitro study, Schaefer D et al. dynamically seeded the fibrous, non-woven PGA meshes and PLGA/PEG foams with chondrocytes and periosteal cells, respectively, (Figures 5C,D). Then, the two cell-polymer constructs were cultured independently and sutured together for an additional co-culture in osteogenic medium. Mature bone-like constructs in combination with immature cartilaginous constructs were suggested to promote integration at the tissue interface. Seo JP et al. inserted PRP/acidic GT sponges loaded with chondrocytes and BMSCs and BMP-2/basic GT sponges loaded with BMSCs into the upper and lower part of osteochondral defects separately in a horse model (Seo et al., 2013). The GT sponges prepared have a porosity of 95.9% with the pore size of 179.1 ± 27.8 μm. Sponges have better mechanical stability compared to meshes. To date, there have been various multi-layered porous scaffolds reported based on different fabricating techniques. Dresing I et al. demonstrated PUR and nHA/PUR scaffolds consisting of three regions (Dresing et al., 2014). The scaffolds were assembled via a solvent welding technique. Jia S et al. designed a biomimetic multi-layered scaffold (Figures 5F). The scaffold comprised three integrated layers: an oriented ACECM-derived cartilage layer, an intermediate compact interfacial layer and a 3DP porous PLGA/TCP layer. The heterogeneous porous constructs provided a suitable template to guide hyaline cartilage, calcified cartilage and subchondral bone growth.
Hydrogel Scaffolds
The extracellular matrix of osteochondral tissue is gelatinous substance containing fibrous components (Khorshidi and Karkhaneh, 2018). Because of the structural and compositional resemblance to natural ECM, natural and synthetic hydrogel scaffolds have great potential in tissue regeneration due to their intrinsic properties, such as biocompatibility, biodegrability and cell interaction. Natural materials used in hydrogels, including decellularized ECM, collagen, chitosan and hyaluronic acid etc., possess satisfactory biocompatibility with no inflammation and cytotoxity, whereas synthetic polymers are easier to process and control (Naahidi et al., 2017). To balance the degradability of scaffolds and the adhesions of cells is the key to design hydrogels for tissue repair. Responsive hydrogel materials are considered as potential candidate for biological platforms due to their adaptive responses to physiological stimuli in the environment. And hydrogel-based soft-hard interfaces, which can regulate homeostasis in the interfacial microenvironment, deserve attention in future studies (Fang et al., 2021).
A biphasic CAN-PAC hydrogel was fabricated by Liao J et al. for osteochondral defect regeneration in a rabbit model (Figures 5G). CSMA and NIPAm were dissolved in the upper solution, meanwhile, PECDA, AAm and PEGDA were added in the lower solution. The hydrogel facilitated the formation of new translucent cartilage and repaired subchondral bone. Also, a hypoxic preconditioned chitosan-based hydrogel has shown a faster healing trend, with thicker cartilage and more new ECM formation (Figures 5H). More recently, Pirosa A et al. used solid gelMA hydrogel in combination with wet-spun PCL and PCL/HA to generate an in vitro vascularized osteochondral tissue model (Figures 5I). In another study, an injectable and self-hardening hydrogel of silylated cellulose and chitosan showed the potential for osteochondral tissue repair in a dog model (Boyer et al., 2020).
Fibrous Scaffolds
Actually, fibrous scaffolds can be classified as porous microfiber or nanofiber scaffolds, which offer a microenvironment favorable for cell attachment and survival. Various materials and techniques can be utilized for fabrications. Electrospinning, in particular, is an widely used technique. Moreover, the fibers can be specifically functionalized by the controlled release of drugs and biomolecules. The application of fibrous scaffolds to osteochondral tissue engineering makes it possible to customize properties as required, including pore morphology, resilience, flexibility and bioactivity etc.
In osteochondral tissue engineering, materials used to produce fibrous scaffolds include natural polymers, synthetic polymers, cellulose fibers, mineral fibers and carbon fibers (Hild et al., 2011; Yunos et al., 2013; Baah-Dwomoh et al., 2015). Liu S et al. produced a nanofiber yarn-collagen type I/hyaluronate hybrid (Yarn-CH)/TCP biphasic scaffold for osteochondral defect repair in a rabbit model. The nanofibrous scaffold showed an almost smooth articulating surface and good integration of cartilage-implant interface. A bilayered microporous scaffold was fabricated with collagen and electrospun poly-L-lactic acid nanofibers (COL-nanofibers) by Zhang S et al. The study reported more rapid osteogenic differentiation and better cartilage formation induced by the nanofibrous scaffold. Another nanofibrous scaffold, namely XanoMatrix, contains polyethylene terephthalate and cellulose acetate (Bhardwaj and Webster, 2016). With great hydrophobicity, 3D surface area and high tensile strength, the scaffold is a candidate for osteochondral defect treatment. Elangomannan S et al. evaluated the properties of carbon nanofibers/PCL/mineralized hydroxyapatite (CNF/PCL/M-HAP) scaffolds and reported the potential for orthopedic applications (Elangomannan et al., 2017).
Microsphere Scaffolds
As an important candidate as building blocks of scaffolds, microspheres (MSs) show advantages in the following respects: biomimetic structural support, biological regulation, controlled release of biomolecules and drugs, cell delivery in vivo and large scale production (He et al., 2020). According to the purpose, scaffolds loaded with MSs come in two types: MS-leached scaffolds and MS-incorporated scaffolds (Figure 6).
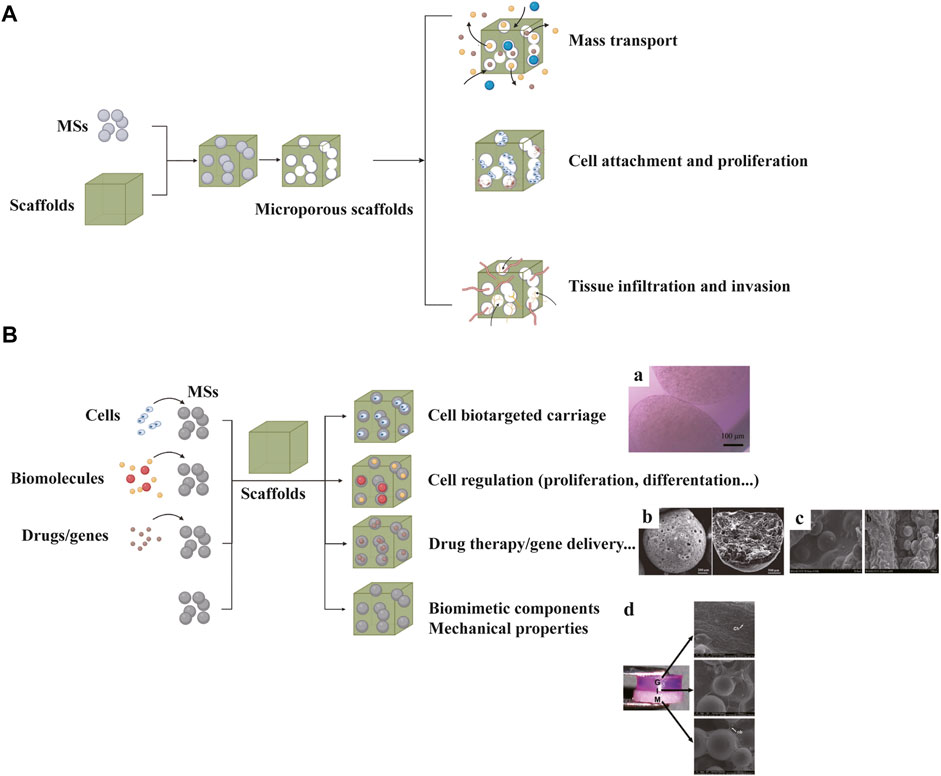
FIGURE 6. Microsphere (MS) scaffolds can be classified into MS-leached scaffolds (A) and MS-incorporated scaffolds (B). Chondrocyte-encapsulated MSs can provide protection and achieve targeted carriage (B: a). MS scaffolds loaded with vancomycin hydrochloride (VH) can reduce inflammation after implantation (B: b). MS scaffolds loaded with OIC-A006 (B: c). Multi-layered scaffold consisting of pre-integrated hydrogel (G), hydrogel + microsphere interface (I) and microsphere (M) bone layers (B: d). (B-a) Adapted with permission from ref (Bozkurt et al., 2019). Copyright 2019 by BioMed Central Ltd. (B-b) Adapted with permission from ref (He et al., 2021). Copyright 2020 by Elsevier Ltd. (B-c) Adapted with permission from ref (Lin et al., 2016). Copyright 2016 by Taylor and Francis Group. (B-d) Adapted with permission from ref (Jiang et al., 2010). Copyright 2010 by Biomedical Engineering Society.
In the former type, MSs serve as porogens to produce homeostatic and regular porous structure. Several studies have reported better cell infiltration and tissue invasion based on MS-leached scaffolds (Zaborowska et al., 2010; Huebsch et al., 2015). In the latter type, MSs are used to modify sophisticated biological functions, thereby showing promise for development of osteochondral tissue engineering. MSs based on hydroxyapatite, calcium carbonate, chitosan and PLGA etc. have been reported to use for scaffold fabrications to improve mechanical strength and bioactivity (Shalumon et al., 2016). Cell-encapsulated MSs can protect the inner cells and allow bidirectional substance exchange (Bozkurt et al., 2019). Moreover, MSs loaded with drugs or biomolecules can exert therapeutic effect and regulate cell metabolism (Dormer et al., 2010). Reyes R et al. incorporated MSs loaded with TGF-β1 and BMP-2 into a bilayered scaffold to induce chondrogenic and osteogenic differentiation, separately (Reyes et al., 2014). The microsphere scaffold was reported to induce a high degree of tissue repair based on histological evidence. In addition, one study encapsulated 45S5 bioactive glass particles (BG) in PLGA MSs, which facilitated mineral formation in the interface and bone regions (Jiang et al., 2010). Mohan N et al. fabricated PLGA-CS-NaHCO3 as chondrogenic MSs and PLGA-β-TCP as osteogenic MSs to produce a gradient scaffold (Mohan et al., 2015). Recently, various studies have reported the utility of drug or gene delivery MSs for effective osteochondral tissue regeneration (Lin et al., 2016; Madry et al., 2020; He et al., 2021).
Metal Scaffolds
To date, limited studies on metal scaffolds have been reported. Mrosek EH et al. used trabecular metal/periosteal graft (TMPG) biocomposites to treat osteochondral defects in a sheep model (Mrosek et al., 2016). The metal scaffolds showed excellent bony ingrowth and integration, but failed to promote satisfactory neo-cartilage formation, unfortunately. Another metal worth mentioning is titanium (Ti), a highly corrosion resistant and biocompatible material with superior mechanical properties (Nover et al., 2015). Given the ability to promote cartilage growth and allow bone tissue ingrowth, porous titanium has been proposed as a viable bone-like base material for osteochondral tissue engineering. The mechanical support of subchondral bone has been reported as an essential part in articular cartilage protection (Hu et al., 2021). Therefore, metallic materials have been employed in OC tissue repair as subchondral bone phase. Duan X et al. fabricated a biphasic scaffold composed of type I collagen (Col)/glycosaminoglycan (GAGs) as chondral phase and porous titanium as subchondral phase (Duan et al., 2013). Sing SL et al. combined the two metals mentioned above to develop a biphasic metal scaffold for osteochondral defect repair (Sing et al., 2017). The titanium-tantalum (TiTa)/type I collagen scaffold demonstrated continuous interface between the two phases, the biological functions of which need to be further evaluated. The poor biodegradability and unsatisfactory performance in mimicking articular cartilage tissue have been the major obstacles to widespread application of metals (Díaz Lantada et al., 2016).
Composite Scaffolds
The use of composite scaffolds in tissue engineering can integrate the advantages of polymers and ceramics. Various bioceramics have been used for scaffold fabrication, mainly summarized into three categories including bioinert materials (e.g., alumina and zirconia), semi-inert surface reactive materials (e.g., bioactive glass and dense hydroxyapatite) and biodegradable materials (e.g., calcium phosphate and tricalcium phosphate) (Pina et al., 2018). Unlike polymers, ceramics have better mechanical stiffness and corrosion resistance. However, several inherent features, such as fragility, inelasticity and difficult processing, limit their applications. Therefore, composite scaffolds made of polymers and ceramics perform better in mechanical properties and degradation behaviors. Erickson AE et al. presented a bilayered scaffold composed of two areas (Erickson et al., 2019) (Figures 7A,B). The cartilage and bone layers contain chitosan/hyaluronic acid (HA) and chitosan/alginate/hydroxyapatite nanorod (HAp), respectively. By the thermally-induced phase separation process, a gradient transition zone between the two layers was established, improving the whole stability. A bilayered chitosan/chitosan-β-tricalcium phosphate (CS/CS-β-TCP) composite scaffold was fabricated and evaluated in a rat model (Xu et al., 2021) (Figure 7C). Cells seeded in both layers maintained excellent viability and differentiated into chondrogenic and osteogenic lineages, respectively. As one of the major projects in designing OC tissue engineering scaffolds, the bone-cartilage interface can regulate the mechanical properties of the composite structure and enhance the integration as a connecting medium. Meanwhile, the interface can inhibit unexpected infiltration and provide independent microenvironment for differentiation. For instance, a four-layered composite scaffold was designed to improve the interfacial bonding between the newly formed tissues and the integration of implants with host tissues (Figure 7D). In the study by Khader A et al., fibrous zinc oxide (ZnO)/PCL composite scaffolds were fabricated and evaluated in vitro (Khader and Arinzeh, 2020). Different percentage ZnO composite scaffolds demonstrated chondrogenic and osteogenic differentiations in different degrees. Bioactive glass (BG) is another important material to produce polymer-bioceramic composite scaffolds (Yao et al., 2014). Due to its osteogenic potential, BG has a remarkable application prospect in osteochondral defect repair. All these studies emphasize the value of composite scaffolds in effective osteochondral tissue engineering. At present, the main challenges in designing this type of scaffolds lie in the balance among stability, mechanical stiffness, biocompatibility, bioactivity and degradability.
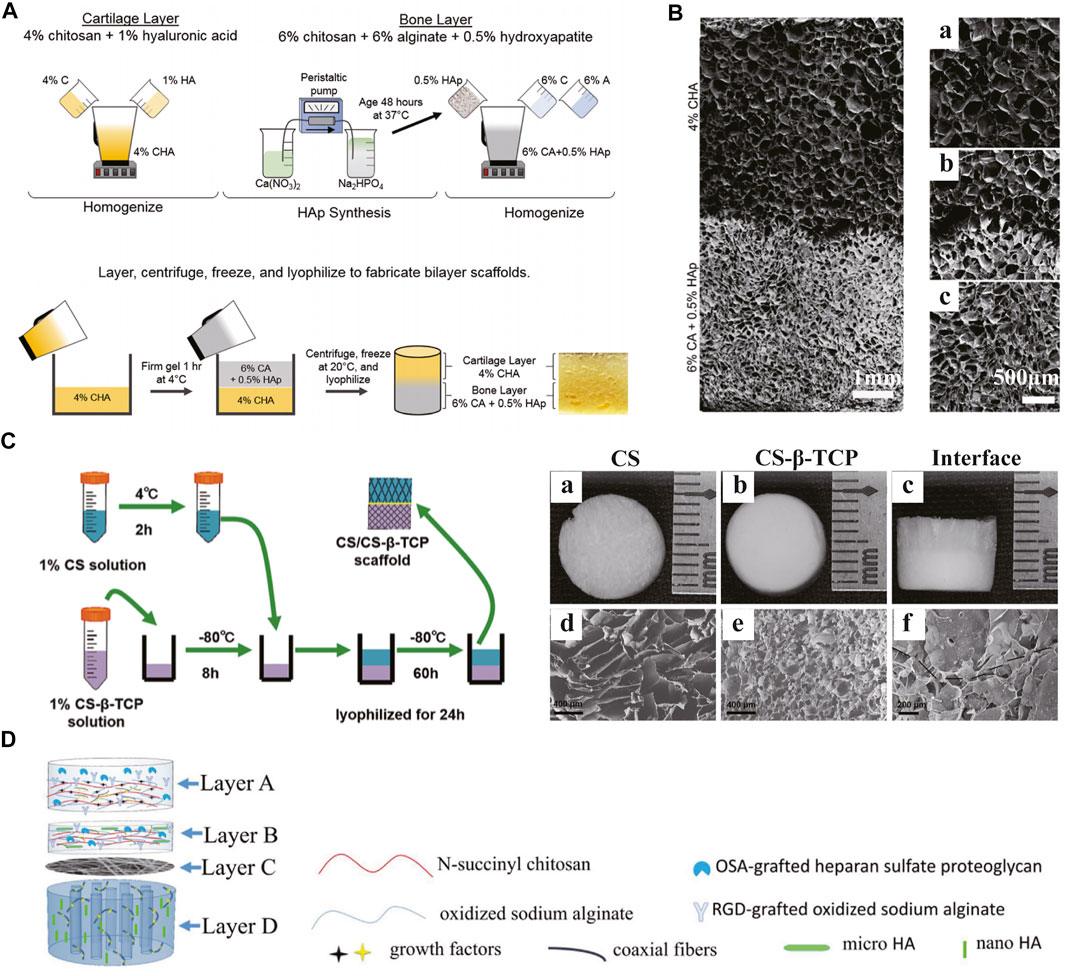
FIGURE 7. Composite scaffolds. The fabrication process (A) and SEM images (B) of a bilayered composite scaffold consisting of 4% CHA cartilage layer (B: a) and 6% CA + 0.5%HAp bone layer (B: c) with an integrated interface (B: b). A bilayered CS/CS-β-TCP scaffold (C) was prepared and observed by gross appearance (C: a–c) and SEM (C: d–f). A composite scaffold with four functional layers improved the integration of implants with host tissues (D). (A and B) Adapted with permission from ref (Erickson et al., 2019). Copyright 2019 by Springer Science + Business Media LLC. (C) Adapted with permission from ref (Xu et al., 2021). Copyright 2021 by Mary Ann Liebert Inc. (D) Adapted with permission from ref (Chen et al., 2018). Copyright 2018 by Elsevier B.V.
Extracellular Matrix-Based Scaffolds
In consideration of the difficulty in mimicking comparable components and complicated architecture of native extracellular matrix (ECM), decellularization of specific tissues, by removing cells and genetic molecules and maintaining biochemical and biomechanical properties, is a potential candidate for scaffold fabrication in osteochondral tissue engineering (Hussey et al., 2018; Yin et al., 2018). Decellularized matrix derived from different tissues, such as urinary bladder, pericardium, dermis and cartilage etc., has been developed and investigated in vitro and in vivo (Singh et al., 2018; Bock et al., 2020). However, decellularized extracellular matrix (dECM), processed to serve as a whole scaffold, has several limitations. Due to the differences in tissue sources, separation and processing techniques and sterilization methods, decellularized scaffolds obtained have certain differences in structures and functions, limiting the reproducibility. Furthermore, unseeded scaffolds promote the natural rebuilding of host tissues, possibly resulting in poor tissue replacement and undesired inogenesis. Efforts should be made to control the potential negative outcomes. Besides, the recellularization of dECM-based scaffolds to mimic native cell distribution is another challenge, considering cell type and concentration, seeding routes and methods and bioreaction characteristics (Hussey et al., 2018). Recently, studies on exosomes possibly pave a new way for OC tissue engineering strategies. Jiang S et al. applied cartilage dECM in combination with intra-articular injection of MSC derived exosomes for osteochondral tissue regeneration (Jiang et al., 2021).
Clinical Products
To date, several synthetic scaffolds applied to osteochondral lesions have been translated into commercial products, some of which have been approved in the European Union, including TruFit™, MaioRegen™, ChondroMimetic®, BioMatrix CRD and Agili-C™ (Wang et al., 2020; D'Ambrosi et al., 2019; Getgood et al., 2012; Kon et al., 2016) (Table 3). Although these bilayered and multilayered scaffolds have reported considerable improvement in clinical symptoms and activity quality, several problems in clinical trials remain to be solved, such as the delamination of scaffolds, the incomplete integration with native tissue, the unsatisfactory quality of regenerated tissue, knee discomfort and the risk of reoperation etc. (Hindle et al., 2014; Brix et al., 2016; Farr et al., 2016; Azam et al., 2018). Therefore, further efforts are needed to illustrate the relationship between these problems and scaffold design strategies for volume production and clinical application.
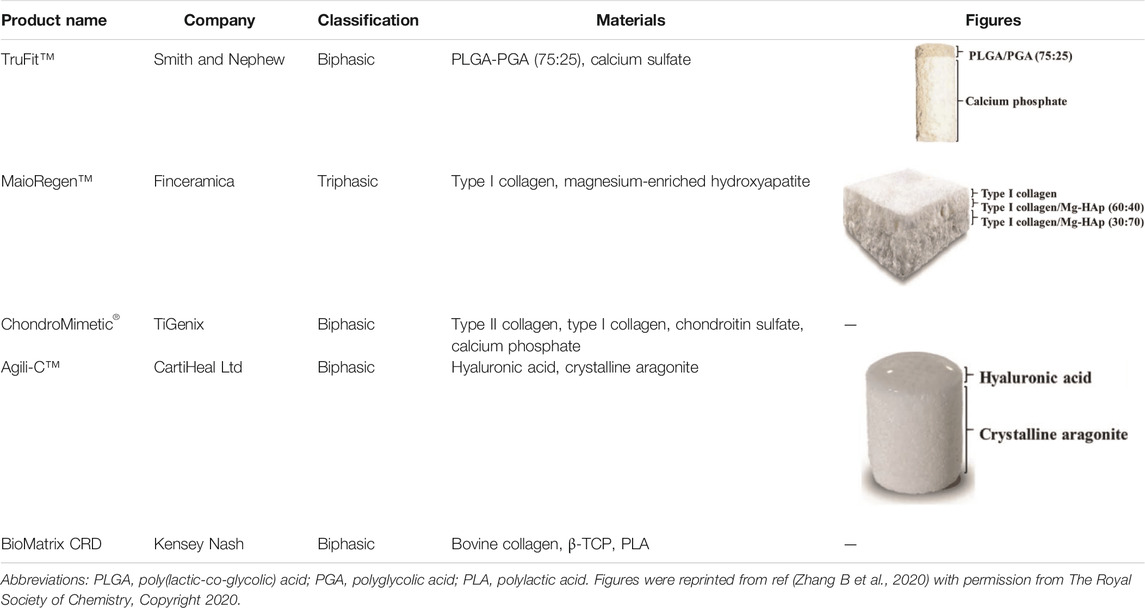
TABLE 3. Hierarchical commercial products for OC tissue regeneration approved in the European Union.
Current Challenges and Future Prospects
Due to the native complexity of OC tissue in hierarchical structure and biological functions, there are still several drawbacks and challenges remaining to be overcome, such as scaffold material-cell interactions, controlled cellular proliferation and differentiation, vascularization and long-term survival of the regenerated tissue etc. Chondrocytes are decisive to the maintenance of the structure and functions of cartilage tissue and can be seeded into scaffolds to produce cartilaginous extracellular matrix (ECM) (Malda et al., 2019). Considering the limitations of chondrocytes, such as isolation difficulty, low proliferation capacity, unexpected dedifferentiation and phenotypic instability, stem cells have offered a new kind of way for OC tissue regeneration. The respective chondrogenic and osteoblastic differentiation of stem cells depends on independent microenvironments, with the assistance of biological or physical stimulations or combined applications (Xuan et al., 2020; Paggi et al., 2020). The spatio-temporal control of stem cell differentiation is an important direction for further research. Due to the low survival rate of cells and instability of bioactive molecules, cells or growth factors incorporated scaffolds are difficult to store and transport, which is an obstacle to commercialization of bioactive scaffolds (Deng et al., 2018). Scaffolds in combination with multiple bioactive ions provide potential directions (Deng et al., 2018) (Figure 8A). Despite the hypothesis that MSCs can differentiate into chondrocytes to regenerate damaged tissue, their secretory functions have attracted more attention recently in tissue repair. Exosomes are possibly candidate for OC tissue engineering (Zhang et al., 2018). Besides, since cells and fibers in native OC tissue have gradient arrangements, the effects of filament orientations and constructs on cellular functions and tissue regeneration should be investigated (Zhang B et al., 2020). Moreover, the integration of scaffolds and regenerating tissue to surrounding targeted tissue is another consistent improving direction, determining the biomechanical properties of the composite structure (F. Zhou et al., 2018). Several strategies, including tissue adhesive and chemical modification, have been applied to enhance integration (Han et al., 2018; Zhang J et al., 2020) (Figure 8B). Despite the comprehensive understanding of the composition and structure of native OC tissue, the reconstruction of its anatomic and biomechanical gradients still remains difficult, which affects the long-term survival of newly formed tissue during joint movements. In comparison to the early developed monophasic scaffolds, biphasic scaffolds can achieve the regeneration of two individual layers, respectively, and triphasic scaffolds can better resemble the OC tissue stratification by taking intermediate CC layer into account. And recently proposed gradient scaffolds, whether in multiphasic or continuous forms, can better imitate the native biochemical components and architectural properties of OC tissue in a more superior way (Wei and Dai, 2021). As a potential direction in future studies, the development of sophisticated gradient OC tissue engineering scaffolds needs more exploration with the assistance of evolving biomaterials and fabrication techniques. Finally, good manufacturing practices and regulatory issues before and after clinical approval are critical to produce safe and efficacious commercial products (Zhou et al., 2020).
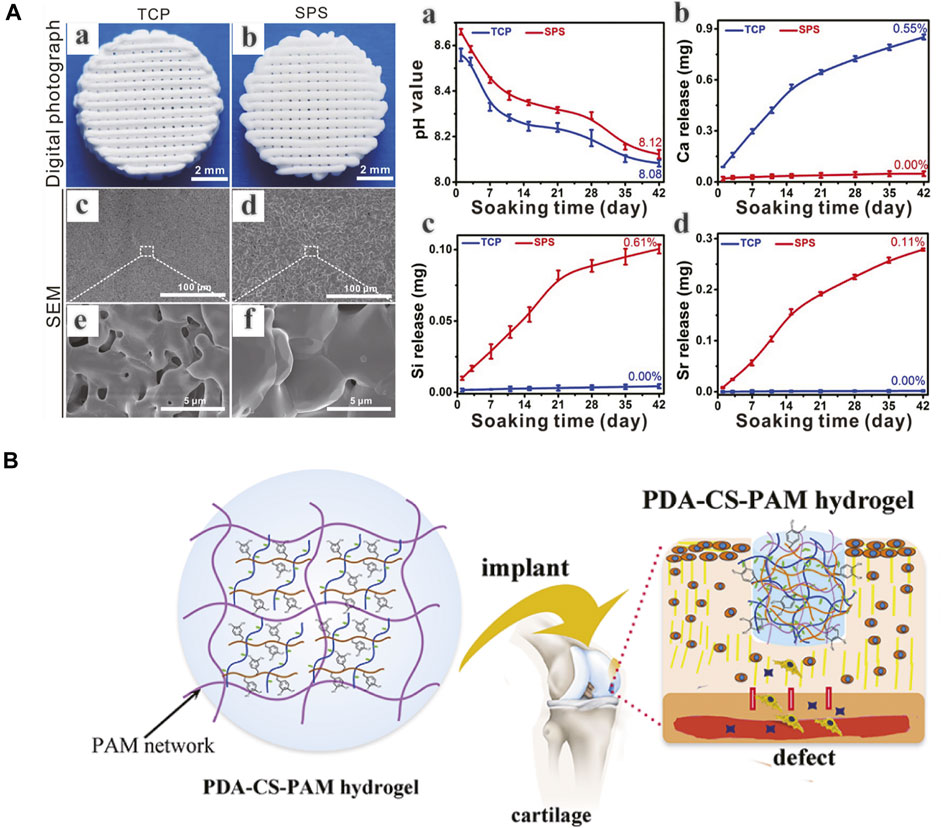
FIGURE 8. (A) 3D-printed Sr5(PO4)2SiO4 (SPS) bioactive ceramic scaffolds can promote osteochondral defect reconstruction possibly by releasing Sr and Si ions (a-d). (B) A polydopamine−chondroitin sulfate−polyacrylamide (PDA−CS−PAM) hydrogel with tissue adhesiveness and super mechanical properties for cartilage regeneration. (A) Adapted with permission from ref (Deng et al., 2018). Copyright 2018 by Ivyspring International Publisher. (B) Adapted with permission from ref (Han et al., 2018). Copyright 2018 by American Chemical Society.
Conclusion
Over the past few decades, osteochondral tissue engineering strategies based on scaffolds have achieved great progress. However, several problems still remain in the restoration of anatomical, biochemical and biomechanical stratification, including the efficiency of cells and bioactive molecules, the integration to adjacent tissues, the spatiotemporal control of physicochemical properties and cellular behaviors and the survival of regenerated tissue etc. In spite of all these challenges, we are optimistic that the development of closely related disciplines and further researches will gradually provide more opportunities for osteochondral tissue engineering for the foreseeable future.
Author Contributions
J-NF, XW, and MY drafted the manuscript. Y-RC, J-YZ, R-HD, and Z-NZ collected and sorted the information. J-KY and F-ZY designed the conception and revised the manuscript. All the authors contributed to the article and approved the submitted version.
Funding
This research was funded by the National Natural Science Foundation of China (51920105006, 51773004, 52003008, and 51973226).
Conflict of Interest
The authors declare that the research was conducted in the absence of any commercial or financial relationships that could be construed as a potential conflict of interest.
Publisher’s Note
All claims expressed in this article are solely those of the authors and do not necessarily represent those of their affiliated organizations, or those of the publisher, the editors and the reviewers. Any product that may be evaluated in this article, or claim that may be made by its manufacturer, is not guaranteed or endorsed by the publisher.
Supplementary Material
The Supplementary Material for this article can be found online at: https://www.frontiersin.org/articles/10.3389/fbioe.2021.812383/full#supplementary-material
Figure S1 | The osteochondral (OC) tissue was observed with Safranin-O/Fast green staining in longitudinal (A) and cross (B) sections. And 3D-model of hyaline cartilage, calcified cartilage zone and subchondral bone was reconstructed. The lower interface of tidemark (C), the upper interface of tidemark (D), the upper interface of cement line (E) and the lower interface of cement line (F) were shown respectively. Scale bar: 200 μm. HC, hyaline cartilage; CCZ, calcified cartilage zone; SB, subchondral bone; TM, tidemark; CL, cement line. Adapted with permission from ref (Wang F et al., 2009). Copyright 2009 by Elsevier Inc.
References
Abarrategi, A., Gutiérrez, M. C., Moreno-Vicente, C., Hortigüela, M. J., Ramos, V., López-Lacomba, J. L., et al. (2008). Multiwall Carbon Nanotube Scaffolds for Tissue Engineering Purposes. Biomaterials 29, 94–102. doi:10.1016/j.biomaterials.2007.09.021
Aguirre-Chagala, Y. E., Altuzar, V., León-Sarabia, E., Tinoco-Magaña, J. C., Yañez-Limón, J. M., and Mendoza-Barrera, C. (2017). Physicochemical Properties of Polycaprolactone/collagen/elastin Nanofibers Fabricated by Electrospinning. Mater. Sci. Eng. C 76, 897–907. Epub 2017 Mar 16. doi:10.1016/j.msec.2017.03.118
Algul, D., Sipahi, H., Aydin, A., Kelleci, F., Ozdatli, S., and Yener, F. G. (2015). Biocompatibility of Biomimetic Multilayered Alginate-Chitosan/β-TCP Scaffold for Osteochondral Tissue. Int. J. Biol. Macromolecules 79, 363–369. Epub 2015 May 14. doi:10.1016/j.ijbiomac.2015.05.005
Ansari, S., Khorshidi, S., and Karkhaneh, A. (2019). Engineering of Gradient Osteochondral Tissue: From Nature to Lab. Acta Biomater. 87, 41–54. Epub 2019 Feb 2. doi:10.1016/j.actbio.2019.01.071
Armiento, A. R., Stoddart, M. J., Alini, M., and Eglin, D. (2018). Biomaterials for Articular Cartilage Tissue Engineering: Learning from Biology. Acta Biomater. 65, 1–20. Epub 2017 Nov 8. doi:10.1016/j.actbio.2017.11.021
Azam, A., Forster, M., and Robertson, A. (2018). Clinical and Radiological Outcome for Trufit Plug in the Treatment of Chondral and Osteochondral Lesions at a Minimum of 2 Years. J. Orthopaedics 15, 47–51. doi:10.1016/j.jor.2018.01.001
Baah-Dwomoh, A., Rolong, A., Gatenholm, P., and Davalos, R. V. (2015). The Feasibility of Using Irreversible Electroporation to Introduce Pores in Bacterial Cellulose Scaffolds for Tissue Engineering. Appl. Microbiol. Biotechnol. 99, 4785–4794. Epub 2015 Feb 18. doi:10.1007/s00253-015-6445-0
Bajek, A., Olkowska, J., Gurtowska, N., Kloskowski, T., Walentowicz-Sadlecka, M., Sadlecki, P., et al. (2014). Human Amniotic-Fluid-Derived Stem Cells: a Unique Source for Regenerative Medicine. Expert Opin. Biol. Ther. 14, 831–839. Epub 2014 Mar 22. doi:10.1517/14712598.2014.898749
Baksh, D., Yao, R., and Tuan, R. S. (2007). Comparison of Proliferative and Multilineage Differentiation Potential of Human Mesenchymal Stem Cells Derived from Umbilical Cord and Bone Marrow. Stem Cells 25, 1384–1392. Epub 2007 Mar 1. doi:10.1634/stemcells.2006-0709
Barrett, A. N., Fong, C.-Y., Subramanian, A., Liu, W., Feng, Y., Choolani, M., et al. (2019). Human Wharton's Jelly Mesenchymal Stem Cells Show Unique Gene Expression Compared with Bone Marrow Mesenchymal Stem Cells Using Single-Cell RNA-Sequencing. Stem Cells Dev. 28, 196–211. Epub 2019 Jan 7. doi:10.1089/scd.2018.0132
Baumgartner, W., Otto, L., Hess, S. C., Stark, W. J., Märsmann, S., Bürgisser, G. M., et al. (2019). Cartilage/bone Interface Fabricated under Perfusion: Spatially Organized Commitment of Adipose‐derived Stem Cells without Medium Supplementation. J. Biomed. Mater. Res. 107, 1833–1843. Epub 2018 Nov 21. doi:10.1002/jbm.b.34276
Bhardwaj, G., and Webster, T. (2016). Enhanced Chondrocyte Culture and Growth on Biologically Inspired Nanofibrous Cell Culture Dishes. Ijn 11, 479–483. doi:10.2147/IJN.S94000
Blair, H. C., Larrouture, Q. C., Li, Y., Lin, H., Beer-Stoltz, D., Liu, L., et al. (2017). Osteoblast Differentiation and Bone Matrix Formation In Vivo and In Vitro. Tissue Eng. B: Rev. 23, 268–280. Epub 2016 Dec 27. doi:10.1089/ten.TEB.2016.0454
Bock, N., Pham, T. L.-B., Nguyen, T. B., Nguyen, T. B., Tran, H. A., and Tran, P. A. (2020). Polydopamine Coating of Uncrosslinked Chitosan as an Acellular Scaffold for Full Thickness Skin Grafts. Carbohydr. Polym. 245, 116524. Epub 2020 Jun 3. doi:10.1016/j.carbpol.2020.116524
Boyer, C., Réthoré, G., Weiss, P., d’Arros, C., Lesoeur, J., Vinatier, C., et al. (2020). A Self-Setting Hydrogel of Silylated Chitosan and Cellulose for the Repair of Osteochondral Defects: From In Vitro Characterization to Preclinical Evaluation in Dogs. Front. Bioeng. Biotechnol. 8, 23. doi:10.3389/fbioe.2020.00023
Bozkurt, M., Aşık, M. D., Gürsoy, S., Türk, M., Karahan, S., Gümüşkaya, B., et al. (2019). Autologous Stem Cell-Derived Chondrocyte Implantation with Bio-Targeted Microspheres for the Treatment of Osteochondral Defects. J. Orthop. Surg. Res. 14, 394. doi:10.1186/s13018-019-1434-0
Brix, M., Kaipel, M., Kellner, R., Schreiner, M., Apprich, S., Boszotta, H., et al. (2016). Successful Osteoconduction but Limited Cartilage Tissue Quality Following Osteochondral Repair by a Cell-free Multilayered Nano-Composite Scaffold at the Knee. Int. Orthopaedics (Sicot) 40, 625–632. Epub 2016 Jan 23. doi:10.1007/s00264-016-3118-2
Burr, D. B., and Gallant, M. A. (2012). Bone Remodelling in Osteoarthritis. Nat. Rev. Rheumatol. 8, 665–673. doi:10.1038/nrrheum.2012.130
Camci-Unal, G., Alemdar, N., Annabi, N., and Khademhosseini, A. (2013). Oxygen-releasing Biomaterials for Tissue Engineering. Polym. Int. 62, 843–848. doi:10.1002/pi.4502
Cao, T., Ho, K. H., and Teoh, S. H. (2003). Scaffold Design and In Vitro Study of Osteochondral Coculture in a Three-Dimensional Porous Polycaprolactone Scaffold Fabricated by Fused Deposition Modeling. Tissue Eng. 9, S103–S112. doi:10.1089/10763270360697012
Carballo, C. B., Nakagawa, Y., Sekiya, I., and Rodeo, S. A. (2017). Basic Science of Articular Cartilage. Clin. Sports Med. 36, 413–425. Epub 2017 Apr 26. doi:10.1016/j.csm.2017.02.001
Chaves, C., Alshomer, F., Palgrave, R. G., and Kalaskar, D. M. (2016). Plasma Surface Modification of Polyhedral Oligomeric Silsequioxane-Poly(carbonate-Urea) Urethane with Allylamine Enhances the Response and Osteogenic Differentiation of Adipose-Derived Stem Cells. ACS Appl. Mater. Inter. 8, 18701–18709. Epub 2016 Jul 15. doi:10.1021/acsami.6b05788
Chen, C., Tambe, D. T., Deng, L., and Yang, L. (2013). Biomechanical Properties and Mechanobiology of the Articular Chondrocyte. Am. J. Physiology-Cell Physiol. 305, C1202–C1208. Epub 2013 Sep 25. doi:10.1152/ajpcell.00242.2013
Chen, M.-Y., Lie, P.-C., Li, Z.-L., and Wei, X. (2009). Endothelial Differentiation of Wharton's Jelly-Derived Mesenchymal Stem Cells in Comparison with Bone Marrow-Derived Mesenchymal Stem Cells. Exp. Hematol. 37, 629–640. doi:10.1016/j.exphem.2009.02.003
Chen, P., Tao, J., Zhu, S., Cai, Y., Mao, Q., Yu, D., et al. (2015). Radially Oriented Collagen Scaffold with SDF-1 Promotes Osteochondral Repair by Facilitating Cell Homing. Biomaterials 39, 114–123. Epub 2014 Nov 15. doi:10.1016/j.biomaterials.2014.10.049
Chen, P., Zheng, L., Wang, Y., Tao, M., Xie, Z., Xia, C., et al. (2019). Desktop-stereolithography 3D Printing of a Radially Oriented Extracellular Matrix/mesenchymal Stem Cell Exosome Bioink for Osteochondral Defect Regeneration. Theranostics 9, 2439–2459. doi:10.7150/thno.31017
Chen, T., Bai, J., Tian, J., Huang, P., Zheng, H., and Wang, J. (2018). A Single Integrated Osteochondral In Situ Composite Scaffold with a Multi-Layered Functional Structure. Colloids Surf. B: Biointerfaces 167, 354–363. Epub 2018 Apr 16. doi:10.1016/j.colsurfb.2018.04.029
Cheng, A., Schwartz, Z., Kahn, A., Li, X., Shao, Z., Sun, M., et al. (2019). Advances in Porous Scaffold Design for Bone and Cartilage Tissue Engineering and Regeneration. Tissue Eng. Part B: Rev. 25, 14–29. Epub 2018 Sep 20. doi:10.1089/ten.TEB.2018.0119
Coryell, P. R., Diekman, B. O., and Loeser, R. F. (2021). Mechanisms and Therapeutic Implications of Cellular Senescence in Osteoarthritis. Nat. Rev. Rheumatol. 17, 47–57. Epub 2020 Nov 18. doi:10.1038/s41584-020-00533-7
Costantini, M., Colosi, C., Mozetic, P., Jaroszewicz, J., Tosato, A., Rainer, A., et al. (2016). Correlation between Porous Texture and Cell Seeding Efficiency of Gas Foaming and Microfluidic Foaming Scaffolds. Mater. Sci. Eng. C 62, 668–677. Epub 2016 Feb 5. doi:10.1016/j.msec.2016.02.010
Cross, L. M., Thakur, A., Jalili, N. A., Detamore, M., and Gaharwar, A. K. (2016). Nanoengineered Biomaterials for Repair and Regeneration of Orthopedic Tissue Interfaces. Acta Biomater. 42, 2–17. Epub 2016 Jun 17. doi:10.1016/j.actbio.2016.06.023
Da, H., Jia, S.-J., Meng, G.-L., Cheng, J.-H., Zhou, W., Xiong, Z., et al. (2013). The Impact of Compact Layer in Biphasic Scaffold on Osteochondral Tissue Engineering. PLoS One 8, e54838. Epub 2013 Jan 28. doi:10.1371/journal.pone.0054838
D’Ambrosi, R., Valli, F., De Luca, P., Ursino, N., and Usuelli, F. (2019). MaioRegen Osteochondral Substitute for the Treatment of Knee Defects: A Systematic Review of the Literature. Jcm 8, 783. doi:10.3390/jcm8060783
Degirmenci, E., Ozturan, K. E., Sahin, A. A., Yilmaz, F., and Kaya, Y. E. (2019). Effects of Tranexamic Acid on the Recovery of Osteochondral Defects Treated by Microfracture and Acellular Matrix Scaffold: an Experimental Study. J. Orthop. Surg. Res. 14, 105. doi:10.1186/s13018-019-1144-7
Dehghani Nazhvani, F., Mohammadi Amirabad, L., Azari, A., Namazi, H., Hosseinzadeh, S., Samanipour, R., et al. (2021). Effects of In Vitro Low Oxygen Tension Preconditioning of Buccal Fat Pad Stem Cells on In Vivo Articular Cartilage Tissue Repair. Life Sci. 280, 119728. Epub 2021 Jun 16. doi:10.1016/j.lfs.2021.119728
Deng, C., Chang, J., and Wu, C. (2019). Bioactive Scaffolds for Osteochondral Regeneration. J. Orthopaedic Translation 17, 15–25. doi:10.1016/j.jot.2018.11.006
Deng, C., Zhu, H., Li, J., Feng, C., Yao, Q., Wang, L., et al. (2018). Bioactive Scaffolds for Regeneration of Cartilage and Subchondral Bone Interface. Theranostics 8, 1940–1955. doi:10.7150/thno.23674
Di Luca, A., Van Blitterswijk, C., and Moroni, L. (2015). The Osteochondral Interface as a Gradient Tissue: from Development to the Fabrication of Gradient Scaffolds for Regenerative Medicine. Birth Defect Res. C 105, 34–52. Epub 2015 Mar 16. doi:10.1002/bdrc.21092
Díaz Lantada, A., Alarcón Iniesta, H., and García-Ruíz, J. P. (2016). Composite Scaffolds for Osteochondral Repair Obtained by Combination of Additive Manufacturing, Leaching Processes and hMSC-CM Functionalization. Mater. Sci. Eng. C 59, 218–227. Epub 2015 Oct 8. doi:10.1016/j.msec.2015.10.015
Ding, C., Qiao, Z., Jiang, W., Li, H., Wei, J., Zhou, G., et al. (2013). Regeneration of a Goat Femoral Head Using a Tissue-specific, Biphasic Scaffold Fabricated with CAD/CAM Technology. Biomaterials 34, 6706–6716. Epub 2013 Jun 14. doi:10.1016/j.biomaterials.2013.05.038
Ding, J., Chen, B., Lv, T., Liu, X., Fu, X., Wang, Q., et al. (2016). Bone Marrow Mesenchymal Stem Cell‐Based Engineered Cartilage Ameliorates Polyglycolic Acid/Polylactic Acid Scaffold‐Induced Inflammation through M2 Polarization of Macrophages in a Pig Model. STEM CELLS Translational Med. 5, 1079–1089. Epub 2016 Jun 8. doi:10.5966/sctm.2015-0263
Dormer, N. H., Singh, M., Wang, L., Berkland, C. J., and Detamore, M. S. (2010). Osteochondral Interface Tissue Engineering Using Macroscopic Gradients of Bioactive Signals. Ann. Biomed. Eng. 38, 2167–2182. Epub 2010 Apr 9. doi:10.1007/s10439-010-0028-0
Dresing, I., Zeiter, S., Auer, J., Alini, M., and Eglin, D. (2014). Evaluation of a Press-Fit Osteochondral Poly(ester-Urethane) Scaffold in a Rabbit Defect Model. J. Mater. Sci. Mater. Med. 25, 1691–1700. Epub 2014 Mar 26. doi:10.1007/s10856-014-5192-6
Duan, P., Pan, Z., Cao, L., He, Y., Wang, H., Qu, Z., et al. (2014). The Effects of Pore Size in Bilayered Poly(lactide-Co-Glycolide) Scaffolds on Restoring Osteochondral Defects in Rabbits. J. Biomed. Mater. Res. 102, 180–192. Epub 2013 May 2. doi:10.1002/jbm.a.34683
Duan, X., Zhu, X., Dong, X., Yang, J., Huang, F., Cen, S., et al. (2013). Repair of Large Osteochondral Defects in a Beagle Model with a Novel Type I Collagen/glycosaminoglycan-Porous Titanium Biphasic Scaffold. Mater. Sci. Eng. C 33, 3951–3957. Epub 2013 May 25. doi:10.1016/j.msec.2013.05.040
Elangomannan, S., Louis, K., Dharmaraj, B. M., Kandasamy, V. S., Soundarapandian, K., and Gopi, D. (2017). Carbon Nanofiber/Polycaprolactone/Mineralized Hydroxyapatite Nanofibrous Scaffolds for Potential Orthopedic Applications. ACS Appl. Mater. Inter. 9, 6342–6355. Epub 2017 Feb 10. doi:10.1021/acsami.6b13058
Erickson, A. E., Sun, J., Lan Levengood, S. K., Swanson, S., Chang, F.-C., Tsao, C. T., et al. (2019). Chitosan-based Composite Bilayer Scaffold as an In Vitro Osteochondral Defect Regeneration Model. Biomed. Microdevices 21, 34. doi:10.1007/s10544-019-0373-1
Erisken, C., Kalyon, D. M., Wang, H., Örnek-Ballanco, C., and Xu, J. (2011). Osteochondral Tissue Formation through Adipose-Derived Stromal Cell Differentiation on Biomimetic Polycaprolactone Nanofibrous Scaffolds with Graded Insulin and Beta-Glycerophosphate Concentrations. Tissue Eng. A 17, 1239–1252. Epub 2011 Feb 3. doi:10.1089/ten.TEA.2009.0693
Fang, Y., Yang, X., Lin, Y., Shi, J., Prominski, A., Clayton, C., et al. (2021). Dissecting Biological and Synthetic Soft-Hard Interfaces for Tissue-like Systems. Chem. Rev. Epub ahead of print. doi:10.1021/acs.chemrev.1c00365
Farooqi, A. R., Bader, R., and van Rienen, U. (2019). Numerical Study on Electromechanics in Cartilage Tissue with Respect to its Electrical Properties. Tissue Eng. Part B: Rev. 25, 152–166. Epub 2018 Dec 31. doi:10.1089/ten.TEB.2018.0214
Farr, J., Gracitelli, G. C., Shah, N., Chang, E. Y., and Gomoll, A. H. (2016). High Failure Rate of a Decellularized Osteochondral Allograft for the Treatment of Cartilage Lesions. Am. J. Sports Med. 44, 2015–2022. Epub. doi:10.1177/0363546516645086
Fell, N. L. A., Lawless, B. M., Cox, S. C., Cooke, M. E., Eisenstein, N. M., Shepherd, D. E. T., et al. (2019). The Role of Subchondral Bone, and its Histomorphology, on the Dynamic Viscoelasticity of Cartilage, Bone and Osteochondral Cores. Osteoarthritis and Cartilage 27, 535–543. Epub 2018 Dec 18. doi:10.1016/j.joca.2018.12.006
Ferguson, V. L., Bushby, A. J., and Boyde, A. (2003). Nanomechanical Properties and mineral Concentration in Articular Calcified Cartilage and Subchondral Bone. J. Anat. 203, 191–202. doi:10.1046/j.1469-7580.2003.00193.x
Florencio-Silva, R., Sasso, G. R. d. S., Sasso-Cerri, E., Simões, M. J., and Cerri, P. S. (2015). Biology of Bone Tissue: Structure, Function, and Factors that Influence Bone Cells. Biomed. Res. Int. 2015, 1–17. Epub 2015 Jul 13. doi:10.1155/2015/421746
Furuya, M., Kikuta, J., Fujimori, S., Seno, S., Maeda, H., Shirazaki, M., et al. (2018). Direct Cell-Cell Contact between Mature Osteoblasts and Osteoclasts Dynamically Controls Their Functions In Vivo. Nat. Commun. 9, 300. doi:10.1038/s41467-017-02541-w
Gao, F., Xu, Z., Liang, Q., Li, H., Peng, L., Wu, M., et al. (2019). Osteochondral Regeneration with 3D‐Printed Biodegradable High‐Strength Supramolecular Polymer Reinforced‐Gelatin Hydrogel Scaffolds. Adv. Sci. 6, 1900867. doi:10.1002/advs.201900867
Gao, P., Jiang, D., Liu, W., Li, H., and Li, Z. (2016). Urine-derived Stem Cells, A New Source of Seed Cells for Tissue Engineering. Cscr 11, 547–553. doi:10.2174/1574888x10666150220161506
Getgood, A., Henson, F., Skelton, C., Herrera, E., Brooks, R., Fortier, L. A., et al. (2012). The Augmentation of a Collagen/Glycosaminoglycan Biphasic Osteochondral Scaffold with Platelet-Rich Plasma and Concentrated Bone Marrow Aspirate for Osteochondral Defect Repair in Sheep. Cartilage 3, 351–363. doi:10.1177/1947603512444597
Gibbs, D. M., Vaezi, M., Yang, S., and Oreffo, R. O. (2014). Hope versus Hype: what Can Additive Manufacturing Realistically Offer Trauma and Orthopedic Surgery? Regenerative Med. 9, 535–549. doi:10.2217/rme.14.20
Goldring, M. B., and Goldring, S. R. (2010). Articular Cartilage and Subchondral Bone in the Pathogenesis of Osteoarthritis. Ann. N. Y Acad. Sci. 1192, 230–237. doi:10.1111/j.1749-6632.2009.05240.x
Goldring, S. R., and Goldring, M. B. (2016). Changes in the Osteochondral Unit during Osteoarthritis: Structure, Function and Cartilage-Bone Crosstalk. Nat. Rev. Rheumatol. 12, 632–644. doi:10.1038/nrrheum.2016.148
Gonçalves, A. M., Moreira, A., Weber, A., Williams, G. R., and Costa, P. F. (2021). Osteochondral Tissue Engineering: The Potential of Electrospinning and Additive Manufacturing. Pharmaceutics 13, 983. doi:10.3390/pharmaceutics13070983
Gracitelli, G. C., Moraes, V. Y., Franciozi, C. E., Luzo, M. V., and Belloti, J. C. (2016). Surgical Interventions (Microfracture, Drilling, Mosaicplasty, and Allograft Transplantation) for Treating Isolated Cartilage Defects of the Knee in Adults. Cochrane Database Syst. Rev. 9, CD010675. doi:10.1002/14651858.CD010675.pub2
Griffin, M. F., Ibrahim, A., Seifalian, A. M., Butler, P. E. M., Kalaskar, D. M., and Ferretti, P. (2017). Chemical Group-dependent Plasma Polymerisation Preferentially Directs Adipose Stem Cell Differentiation towards Osteogenic or Chondrogenic Lineages. Acta Biomater. 50, 450–461. Epub 2016 Dec 9. doi:10.1016/j.actbio.2016.12.016
Guan, J.-J., Niu, X., Gong, F.-X., Hu, B., Guo, S.-C., Lou, Y.-L., et al. (2014). Biological Characteristics of Human-Urine-Derived Stem Cells: Potential for Cell-Based Therapy in Neurology. Tissue Eng. Part A 20, 1794–1806. Epub 2014 May 19. doi:10.1089/ten.TEA.2013.0584
Han, F., Zhou, F., Yang, X., Zhao, J., Zhao, Y., and Yuan, X. (2015). A Pilot Study of Conically Graded Chitosan-Gelatin Hydrogel/PLGA Scaffold with Dual-Delivery of TGF-Β1 and BMP-2 for Regeneration of Cartilage-Bone Interface. J. Biomed. Mater. Res. 103, 1344–1353. Epub 2014 Nov 11. doi:10.1002/jbm.b.33314
Han, L., Wang, M., Li, P., Gan, D., Yan, L., Xu, J., et al. (2018). Mussel-Inspired Tissue-Adhesive Hydrogel Based on the Polydopamine-Chondroitin Sulfate Complex for Growth-factor-free Cartilage Regeneration. ACS Appl. Mater. Inter. 10, 28015–28026. Epub 2018 Aug 10. doi:10.1021/acsami.8b05314
He, J., Hu, X., Cao, J., Zhang, Y., Xiao, J., Peng, l., et al. (2021). Chitosan-coated Hydroxyapatite and Drug-Loaded Polytrimethylene Carbonate/polylactic Acid Scaffold for Enhancing Bone Regeneration. Carbohydr. Polym. 253, 117198. Epub 2020 Oct 10. doi:10.1016/j.carbpol.2020.117198
He, Q., Zhang, J., Liao, Y., Alakpa, E. V., Bunpetch, V., Zhang, J., et al. (2020). Current Advances in Microsphere Based Cell Culture and Tissue Engineering. Biotechnol. Adv. 39, 107459. Epub 2019 Nov 1. doi:10.1016/j.biotechadv.2019.107459
Hild, N., Schneider, O. D., Mohn, D., Luechinger, N. A., Koehler, F. M., Hofmann, S., et al. (2011). Two-layer Membranes of Calcium Phosphate/collagen/PLGA Nanofibres: In Vitro Biomineralisation and Osteogenic Differentiation of Human Mesenchymal Stem Cells. Nanoscale 3, 401–409. Epub 2010 Nov 9. doi:10.1039/c0nr00615g
Hindle, P., Hendry, J. L., Keating, J. F., and Biant, L. C. (2014). Autologous Osteochondral Mosaicplasty or TruFit Plugs for Cartilage Repair. Knee Surg. Sports Traumatol. Arthrosc. 22, 1235–1240. Epub 2013 Apr 16. doi:10.1007/s00167-013-2493-0
Hossain, M. S., Bergstrom, D. J., and Chen, X. B. (2015). Modelling and Simulation of the Chondrocyte Cell Growth, Glucose Consumption and Lactate Production within a Porous Tissue Scaffold inside a Perfusion Bioreactor. Biotechnol. Rep. 5, 55–62. doi:10.1016/j.btre.2014.12.002
Hu, K., and Olsen, B. R. (2016). Osteoblast-derived VEGF Regulates Osteoblast Differentiation and Bone Formation during Bone Repair. J. Clin. Invest. 126, 509–526. doi:10.1172/JCI82585
Hu, Y., Chen, X., Wang, S., Jing, Y., and Su, J. (2021). Subchondral Bone Microenvironment in Osteoarthritis and Pain. Bone Res. 9, 20. doi:10.1038/s41413-021-00147-z
Huebsch, N., Lippens, E., Lee, K., Mehta, M., Koshy, S. T., Darnell, M. C., et al. (2015). Matrix Elasticity of Void-Forming Hydrogels Controls Transplanted-Stem-Cell-Mediated Bone Formation. Nat. Mater 14, 1269–1277. doi:10.1038/nmat4407
Hunter, D. J., and Bierma-Zeinstra, S. (2019). Osteoarthritis. The Lancet 393, 1745–1759. doi:10.1016/S0140-6736(19)30417-9
Hunziker, E. B., Quinn, T. M., and Häuselmann, H.-J. (2002). Quantitative Structural Organization of normal Adult Human Articular Cartilage. Osteoarthritis and Cartilage 10, 564–572. doi:10.1053/joca.2002.0814
Hussey, G. S., Dziki, J. L., and Badylak, S. F. (2018). Extracellular Matrix-Based Materials for Regenerative Medicine. Nat. Rev. Mater. 3, 159–173. doi:10.1038/s41578-018-0023-x
Hutmacher, D. W. (2000). Scaffolds in Tissue Engineering Bone and Cartilage. Biomaterials 21, 2529–2543. doi:10.1016/s0142-9612(00)00121-6
Jacob, J., More, N., Kalia, K., and Kapusetti, G. (2018). Piezoelectric Smart Biomaterials for Bone and Cartilage Tissue Engineering. Inflamm. Regener 38, 2. doi:10.1186/s41232-018-0059-8
Jeon, J. E., Vaquette, C., Theodoropoulos, C., Klein, T. J., and Hutmacher, D. W. (2014). Multiphasic Construct Studied in an Ectopic Osteochondral Defect Model. J. R. Soc. Interf. 11, 20140184. doi:10.1098/rsif.2014.0184
Jia, S., Wang, J., Zhang, T., Pan, W., Li, Z., He, X., et al. (2018). Multilayered Scaffold with a Compact Interfacial Layer Enhances Osteochondral Defect Repair. ACS Appl. Mater. Inter. 10, 20296–20305. Epub 2018 Jun 5. doi:10.1021/acsami.8b03445
Jiang, J., Tang, A., Ateshian, G. A., Guo, X. E., Hung, C. T., and Lu, H. H. (2010). Bioactive Stratified Polymer Ceramic-Hydrogel Scaffold for Integrative Osteochondral Repair. Ann. Biomed. Eng. 38, 2183–2196. Epub 2010 Apr 22. doi:10.1007/s10439-010-0038-y
Jiang, S., Tian, G., Yang, Z., Gao, X., Wang, F., Li, J., et al. (2021). Enhancement of Acellular Cartilage Matrix Scaffold by Wharton's Jelly Mesenchymal Stem Cell-Derived Exosomes to Promote Osteochondral Regeneration. Bioactive Mater. 6, 2711–2728. doi:10.1016/j.bioactmat.2021.01.031
Jiang, Y., Chen, L., Zhang, S., Tong, T., Zhang, W., Liu, W., et al. (2013). Incorporation of Bioactive Polyvinylpyrrolidone-Iodine within Bilayered Collagen Scaffolds Enhances the Differentiation and Subchondral Osteogenesis of Mesenchymal Stem Cells. Acta Biomater. 9, 8089–8098. Epub 2013 May 23. doi:10.1016/j.actbio.2013.05.014
Kagami, H., Agata, H., and Tojo, A. (2011). Bone Marrow Stromal Cells (Bone Marrow-Derived Multipotent Mesenchymal Stromal Cells) for Bone Tissue Engineering: Basic Science to Clinical Translation. Int. J. Biochem. Cell Biol. 43, 286–289. Epub 2010 Dec 13. doi:10.1016/j.biocel.2010.12.006
Kandel, R. A., Grynpas, M., Pilliar, R., Lee, J., Wang, J., Waldman, S., et al. (2006). CIHR-bioengineering of Skeletal Tissues TeamRepair of Osteochondral Defects with Biphasic Cartilage-Calcium Polyphosphate Constructs in a Sheep Model. Biomaterials 27, 4120–4131. Epub 2006 Mar 29. doi:10.1016/j.biomaterials.2006.03.005
Karkhaneh, A., Naghizadeh, Z., Shokrgozar, M. A., Bonakdar, S., Solouk, A., and Haghighipour, N. (2014). Effects of Hydrostatic Pressure on Biosynthetic Activity during Chondrogenic Differentiation of MSCs in Hybrid Scaffolds. Int. J. Artif. Organs 37, 142–148. Epub 2014 Feb 7. doi:10.5301/ijao.5000288
Karner, C. M., and Long, F. (2018). Glucose Metabolism in Bone. Bone 115, 2–7. Epub 2017 Aug 24. doi:10.1016/j.bone.2017.08.008
Khader, A., and Arinzeh, T. L. (2020). Biodegradable Zinc Oxide Composite Scaffolds Promote Osteochondral Differentiation of Mesenchymal Stem Cells. Biotechnol. Bioeng. 117, 194–209. Epub 2019 Oct 6. doi:10.1002/bit.27173
Khorshidi, S., and Karkhaneh, A. (2018). A Review on Gradient Hydrogel/fiber Scaffolds for Osteochondral Regeneration. J. Tissue Eng. Regen. Med. 12, e1974–e1990. Epub 2018 Jan 28. doi:10.1002/term.2628
Kim, H. S., Mandakhbayar, N., Kim, H.-W., Leong, K. W., and Yoo, H. S. (2021). Protein-reactive Nanofibrils Decorated with Cartilage-Derived Decellularized Extracellular Matrix for Osteochondral Defects. Biomaterials 269, 120214. Epub 2020 Jun 27. doi:10.1016/j.biomaterials.2020.120214
Kim, S. H., Kim, S. H., and Jung, Y. (2015). Bi-layered PLCL/(PLGA/β-TCP) Composite Scaffold for Osteochondral Tissue Engineering. J. Bioactive Compatible Polym. 30, 178–187. doi:10.1177/0883911514566015
Kim, T. W., Lee, M. C., Bae, H. C., and Han, H. S. (2018). Direct Coculture of Human Chondrocytes and Synovium-Derived Stem Cells Enhances In Vitro Chondrogenesis. Cell J 20, 53–60. doi:10.22074/cellj.2018.5025
Kim, Y. G., Choi, J., and Kim, K. (2020). Mesenchymal Stem Cell‐Derived Exosomes for Effective Cartilage Tissue Repair and Treatment of Osteoarthritis. Biotechnol. J. 15, 2000082. Epub 2020 Jul 13. doi:10.1002/biot.202000082
Knothe Tate, M. L., Adamson, J. R., Tami, A. E., and Bauer, T. W. (2004). The Osteocyte. Int. J. Biochem. Cell Biol. 36, 1–8. doi:10.1016/s1357-2725(03)00241-3
Komori, T. (2016). Cell Death in Chondrocytes, Osteoblasts, and Osteocytes. Ijms 17, 2045. doi:10.3390/ijms17122045
Komori, T. (2019). Regulation of Proliferation, Differentiation and Functions of Osteoblasts by Runx2. Ijms 20, 1694. doi:10.3390/ijms20071694
Kon, E., Robinson, D., Verdonk, P., Drobnic, M., Patrascu, J. M., Dulic, O., et al. (2016). A Novel Aragonite-Based Scaffold for Osteochondral Regeneration: Early Experience on Human Implants and Technical Developments. Injury 47 (Suppl. 6), S27–S32. doi:10.1016/S0020-1383(16)30836-1
Kreipke, T. C., and Niebur, G. L. (2017). Anisotropic Permeability of Trabecular Bone and its Relationship to Fabric and Architecture: A Computational Study. Ann. Biomed. Eng. 45, 1543–1554. Epub 2017 Feb 2. doi:10.1007/s10439-017-1805-9
Kwon, H., Brown, W. E., Lee, C. A., Wang, D., Paschos, N., Hu, J. C., et al. (2019). Surgical and Tissue Engineering Strategies for Articular Cartilage and Meniscus Repair. Nat. Rev. Rheumatol. 15, 550–570. Epub 2019 Jul 11. doi:10.1038/s41584-019-0255-1
Le, T. M., Vu, N. B., Huynh, P. D., and Van Pham, P. (2021). Treatment of Osteochondral Femoral Head Defect by Human Umbilical Cord Mesenchymal Stem Cell Sheet Transplantation: An Experimental Study in Rats. Adv. Exp. Med. Biol. Epub ahead of print. doi:10.1007/5584_2021_671
Levingstone, T. J., Thompson, E., Matsiko, A., Schepens, A., Gleeson, J. P., and O’Brien, F. J. (2016). Multi-layered Collagen-Based Scaffolds for Osteochondral Defect Repair in Rabbits. Acta Biomater. 32, 149–160. Epub 2015 Dec 24. doi:10.1016/j.actbio.2015.12.034
Li, C., Ouyang, L., Armstrong, J. P. K., and Stevens, M. M. (2021). Advances in the Fabrication of Biomaterials for Gradient Tissue Engineering. Trends Biotechnol. 39, 150–164. Epub 2020 Jul 7. doi:10.1016/j.tibtech.2020.06.005
Liao, J., Tian, T., Shi, S., Xie, X., Ma, Q., Li, G., et al. (2017). The Fabrication of Biomimetic Biphasic CAN-PAC Hydrogel with a Seamless Interfacial Layer Applied in Osteochondral Defect Repair. Bone Res. 5, 17018. doi:10.1038/boneres.2017.18
Lin, L., Wang, T., Zhou, Q., and Qian, N. (2016). The Effects of Different Amounts of Drug Microspheres on the Vivo and Vitro Performance of the PLGA/β-TCP Scaffold. Designed Monomers Polym. 20, 351–362. doi:10.1080/15685551.2016.1259839
Liu, S., Wu, J., Liu, X., Chen, D., Bowlin, G. L., Cao, L., et al. (2015). Osteochondral Regeneration Using an Oriented Nanofiber Yarn-Collagen Type I/hyaluronate Hybrid/TCP Biphasic Scaffold. J. Biomed. Mater. Res. 103, 581–592. Epub 2014 May 7. doi:10.1002/jbm.a.35206
Liu, S., Yuan, M., Hou, K., Zhang, L., Zheng, X., Zhao, B., et al. (2012). Immune Characterization of Mesenchymal Stem Cells in Human Umbilical Cord Wharton's Jelly and Derived Cartilage Cells. Cell Immunol. 278, 35–44. Epub 2012 Jul 16. doi:10.1016/j.cellimm.2012.06.010
Liu, W., Lipner, J., Moran, C. H., Feng, L., Li, X., Thomopoulos, S., et al. (2015). Generation of Electrospun Nanofibers with Controllable Degrees of Crimping through a Simple, Plasticizer-Based Treatment. Adv. Mater. 27, 2583–2588. Epub 2015 Mar 10. doi:10.1002/adma.201500329
Liu, Y.-Y., Yu, H.-C., Liu, Y., Liang, G., Zhang, T., and Hu, Q.-X. (2016). Dual Drug Spatiotemporal Release from Functional Gradient Scaffolds Prepared Using 3D Bioprinting and Electrospinning. Polym. Eng. Sci. 56, 170–177. doi:10.1002/pen.24239
Loeser, R. F. (2014). Integrins and Chondrocyte-Matrix Interactions in Articular Cartilage. Matrix Biol. 39, 11–16. Epub 2014 Aug 25. doi:10.1016/j.matbio.2014.08.007
Lyons, T. J., McClure, S. F., Stoddart, R. W., and McClure, J. (2006). The normal Human Chondro-Osseous Junctional Region: Evidence for Contact of Uncalcified Cartilage with Subchondral Bone and Marrow Spaces. BMC Musculoskelet. Disord. 7, 52. doi:10.1186/1471-2474-7-52
M, J. C., Konwarh, R., Knowles, J. C., and Mandal, B. B. (2017). Mimicking Hierarchical Complexity of the Osteochondral Interface Using Electrospun Silk-Bioactive Glass Composites. ACS Appl. Mater. Inter. 9, 8000–8013. Epub 2017 Feb 22. doi:10.1021/acsami.6b16590
Ma, K., Zhao, T., Yang, L., Wang, P., Jin, J., Teng, H., et al. (2020). Application of Robotic-Assisted In Situ 3D Printing in Cartilage Regeneration with HAMA Hydrogel: An In Vivo Study. J. Adv. Res. 23, 123–132. doi:10.1016/j.jare.2020.01.010
Ma, N., Wang, H., Xu, X., Wan, Y., Liu, Y., Wang, M., et al. (2017). Autologous-cell-derived, Tissue-Engineered Cartilage for Repairing Articular Cartilage Lesions in the Knee: Study Protocol for a Randomized Controlled Trial. Trials 18, 519. doi:10.1186/s13063-017-2251-6
Madry, H., Venkatesan, J. K., Carballo-Pedrares, N., Rey-Rico, A., and Cucchiarini, M. (2020). Scaffold-Mediated Gene Delivery for Osteochondral Repair. Pharmaceutics 12, 930. doi:10.3390/pharmaceutics12100930
Mahmoudifar, N., and Doran, P. M. (2013). Osteogenic Differentiation and Osteochondral Tissue Engineering Using Human Adipose-Derived Stem Cells. Biotechnol. Prog. 29, 176–185. Epub 2012 Dec 20. doi:10.1002/btpr.1663
Maia, F. R., Carvalho, M. R., Oliveira, J. M., and Reis, R. L. (2018). Tissue Engineering Strategies for Osteochondral Repair. Adv. Exp. Med. Biol. 1059, 353–371. doi:10.1007/978-3-319-76735-2_16
Malda, J., Groll, J., and van Weeren, P. R. (2019). Rethinking Articular Cartilage Regeneration Based on a 250-Year-Old Statement. Nat. Rev. Rheumatol. 15, 571–572. doi:10.1038/s41584-019-0278-7
Maleki, M., Hashlamoun, K., Herzog, W., and Federico, S. (2020). Effect of Structural Distortions on Articular Cartilage Permeability under Large Deformations. Biomech. Model. Mechanobiol 19, 317–334. Epub 2019 Sep 10. doi:10.1007/s10237-019-01213-6
Maraldi, T., Riccio, M., Resca, E., Pisciotta, A., La Sala, G. B., Ferrari, A., et al. (2011). Human Amniotic Fluid Stem Cells Seeded in Fibroin Scaffold Produce In Vivo Mineralized Matrix. Tissue Eng. Part A 17, 2833–2843. Epub 2011 Aug 24. doi:10.1089/ten.tea.2011.0062
Mendes, L. F., Katagiri, H., Tam, W. L., Chai, Y. C., Geris, L., Roberts, S. J., et al. (2018). Advancing Osteochondral Tissue Engineering: Bone Morphogenetic Protein, Transforming Growth Factor, and Fibroblast Growth Factor Signaling Drive Ordered Differentiation of Periosteal Cells Resulting in Stable Cartilage and Bone Formation In Vivo. Stem Cell Res Ther 9, 42. doi:10.1186/s13287-018-0787-3
Mohan, N., Gupta, V., Sridharan, B. P., Mellott, A. J., Easley, J. T., Palmer, R. H., et al. (2015). Microsphere-based Gradient Implants for Osteochondral Regeneration: a Long-Term Study in Sheep. Regenerative Med. 10, 709–728. doi:10.2217/rme.15.38
More, N., and Kapusetti, G. (2017). Piezoelectric Material - A Promising Approach for Bone and Cartilage Regeneration. Med. Hypotheses 108, 10–16. Epub 2017 Jul 25. doi:10.1016/j.mehy.2017.07.021
Morgan, E. F., Unnikrisnan, G. U., and Hussein, A. I. (2018). Bone Mechanical Properties in Healthy and Diseased States. Annu. Rev. Biomed. Eng. 20, 119–143. doi:10.1146/annurev-bioeng-062117-121139
Mow, V. C., Ratcliffe, A., and Robin Poole, A. (1992). Cartilage and Diarthrodial Joints as Paradigms for Hierarchical Materials and Structures. Biomaterials 13, 67–97. doi:10.1016/0142-9612(92)90001-5
Mrosek, E. H., Chung, H.-W., Fitzsimmons, J. S., O’Driscoll, S. W., Reinholz, G. G., and Schagemann, J. C. (2016). Porous Tantalum Biocomposites for Osteochondral Defect Repair. Bone Jt. Res. 5, 403–411. doi:10.1302/2046-3758.59.BJR-2016-000.R110.1302/2046-3758.59.bjr-2016-0070.r1
Murata, D., Fujimoto, R., and Nakayama, K. (2020). Osteochondral Regeneration Using Adipose Tissue-Derived Mesenchymal Stem Cells. Ijms 21, 3589. doi:10.3390/ijms21103589
Murphy, L., and Helmick, C. G. (2012). The Impact of Osteoarthritis in the United States. Am. J. Nurs. 112, S13–S19. doi:10.1097/01.NAJ.0000412646.80054.21
Naahidi, S., Jafari, M., Logan, M., Wang, Y., Yuan, Y., Bae, H., et al. (2017). Biocompatibility of Hydrogel-Based Scaffolds for Tissue Engineering Applications. Biotechnol. Adv. 35, 530–544. Epub 2017 May 27. doi:10.1016/j.biotechadv.2017.05.006
Natarajan, A. B. M., Sivadas, V. P. D., and Nair, P. D. P. D. (2021). 3D-printed Biphasic Scaffolds for the Simultaneous Regeneration of Osteochondral Tissues. Biomed. Mater. 16, 054102. doi:10.1088/1748-605X/ac14cb
Nover, A. B., Lee, S. L., Georgescu, M. S., Howard, D. R., Saunders, R. A., Yu, W. T., et al. (2015). Porous Titanium Bases for Osteochondral Tissue Engineering. Acta Biomater. 27, 286–293. Epub 2015 Aug 28. doi:10.1016/j.actbio.2015.08.045
Nukavarapu, S. P., and Dorcemus, D. L. (2013). Osteochondral Tissue Engineering: Current Strategies and Challenges. Biotechnol. Adv. 31, 706–721. Epub 2012 Nov 19. doi:10.1016/j.biotechadv.2012.11.004
Paggi, C. A., Venzac, B., Karperien, M., Leijten, J. C. H., and Le Gac, S. (2020). Monolithic Microfluidic Platform for Exerting Gradients of Compression on Cell-Laden Hydrogels, and Application to a Model of the Articular Cartilage. Sensors Actuators B: Chem. 315, 127917. doi:10.1016/j.snb.2020.127917
Pan, J., Zhou, X., Li, W., Novotny, J. E., Doty, S. B., and Wang, L. (2009). In Situ measurement of Transport between Subchondral Bone and Articular Cartilage. J. Orthop. Res. 27, 1347–1352. doi:10.1002/jor.20883
Pina, S., Rebelo, R., Correlo, V. M., Oliveira, J. M., and Reis, R. L. (2018). Bioceramics for Osteochondral Tissue Engineering and Regeneration. Adv. Exp. Med. Biol. 1058, 53–75. doi:10.1007/978-3-319-76711-6_3
Pirosa, A., Gottardi, R., Alexander, P. G., Puppi, D., Chiellini, F., and Tuan, R. S. (2021). An In Vitro Chondro-Osteo-Vascular Triphasic Model of the Osteochondral Complex. Biomaterials 272, 120773. Epub 2021 Mar 22. doi:10.1016/j.biomaterials.2021.120773
Poole, C. A., Flint, M. H., and Beaumont, B. W. (1987). Chondrons in Cartilage: Ultrastructural Analysis of the Pericellular Microenvironment in Adult Human Articular Cartilages. J. Orthop. Res. 5, 509–522. doi:10.1002/jor.1100050406
Prasanna, S. J., Gopalakrishnan, D., Shankar, S. R., and Vasandan, A. B. (2010). Pro-Inflammatory Cytokines, IFNγ and TNFα, Influence Immune Properties of Human Bone Marrow and Wharton Jelly Mesenchymal Stem Cells Differentially. PLoS One 5, e9016. doi:10.1371/journal.pone.0009016
Qin, H., Zhu, C., An, Z., Jiang, Y., Zhao, Y., Wang, J., et al. (2014). Silver Nanoparticles Promote Osteogenic Differentiation of Human Urine-Derived Stem Cells at Noncytotoxic Concentrations. Ijn 9, 2469–2478. doi:10.2147/IJN.S59753
Raeisdasteh Hokmabad, V., Davaran, S., Ramazani, A., and Salehi, R. (2017). Design and Fabrication of Porous Biodegradable Scaffolds: a Strategy for Tissue Engineering. J. Biomater. Sci. Polym. Edition 28, 1797–1825. doi:10.1080/09205063.2017.1354674
Raucci, M. G., Demitri, C., Soriente, A., Fasolino, I., Sannino, A., and Ambrosio, L. (2018). Gelatin/nano-hydroxyapatite Hydrogel Scaffold Prepared by Sol-Gel Technology as Filler to Repair Bone Defects. J. Biomed. Mater. Res. 106, 2007–2019. Epub 2018 Apr 17. doi:10.1002/jbm.a.36395
Redman, S., Oldfield, S. F., Oldfield, S., and Archer, C. (2005). Current Strategies for Articular Cartilage Repair. eCM 9, 23–32. doi:10.22203/ecm.v009a04
Re’em, T., Witte, F., Willbold, E., Ruvinov, E., and Cohen, S. (2012). Simultaneous Regeneration of Articular Cartilage and Subchondral Bone Induced by Spatially Presented TGF-Beta and BMP-4 in a Bilayer Affinity Binding System. Acta Biomater. 8, 3283–3293. Epub 2012 May 19. doi:10.1016/j.actbio.2012.05.014
Ren, X., Wang, F., Chen, C., Gong, X., Yin, L., and Yang, L. (2016). Engineering Zonal Cartilage through Bioprinting Collagen Type II Hydrogel Constructs with Biomimetic Chondrocyte Density Gradient. BMC Musculoskelet. Disord. 17, 301. doi:10.1186/s12891-016-1130-8
Responte, D. J., Natoli, R. M., and Athanasiou, K. A. (2007). Collagens of Articular Cartilage: Structure, Function, and Importance in Tissue Engineering. Crit. Rev. Biomed. Eng. 35, 363–411. doi:10.1615/critrevbiomedeng.v35.i5.20
Reyes, R., Delgado, A., Solis, R., Sanchez, E., Hernandez, A., Roman, J. S., et al. (2014). Cartilage Repair by Local Delivery of Transforming Growth Factor-Β1 or Bone Morphogenetic Protein-2 from a Novel, Segmented Polyurethane/polylactic-Co -glycolic Bilayered Scaffold. J. Biomed. Mater. Res. 102, 1110–1120. Epub 2013 Jun 14. doi:10.1002/jbma.3476910.1002/jbm.a.34769
Ribeiro, V. P., Pina, S., Costa, J. B., Cengiz, I. F., García-Fernández, L., Fernández-Gutiérrez, M. d. M., et al. (2019). Enzymatically Cross-Linked Silk Fibroin-Based Hierarchical Scaffolds for Osteochondral Regeneration. ACS Appl. Mater. Inter. 11, 3781–3799. Epub 2019 Jan 16. doi:10.1021/acsami.8b21259
Sadtler, K., Singh, A., Wolf, M. T., Wang, X., Pardoll, D. M., and Elisseeff, J. H. (2016). Design, Clinical Translation and Immunological Response of Biomaterials in Regenerative Medicine. Nat. Rev. Mater. 1, 16040. doi:10.1038/natrevmats.2016.40
Salonius, E., Muhonen, V., Lehto, K., Järvinen, E., Pyhältö, T., Hannula, M., et al. (2019). Gas‐foamed Poly(lactide‐co‐glycolide) and Poly(lactide‐co‐glycolide) with Bioactive Glass Fibres Demonstrate Insufficient Bone Repair in Lapine Osteochondral Defects. J. Tissue Eng. Regen. Med. 13, 406–415. Epub 2019 Feb 18. doi:10.1002/term.2801
Sasaki, A., Mizuno, M., Ozeki, N., Katano, H., Otabe, K., Tsuji, K., et al. (2018). Canine Mesenchymal Stem Cells from Synovium Have a Higher Chondrogenic Potential Than Those from Infrapatellar Fat Pad, Adipose Tissue, and Bone Marrow. PLoS One 13, e0202922. doi:10.1371/journal.pone.0202922
Schaefer, D., Martin, I., Shastri, P., Padera, R. F., Langer, R., Freed, L. E., et al. (2000). In Vitro generation of Osteochondral Composites. Biomaterials 21, 2599–2606. doi:10.1016/s0142-9612(00)00127-7
Schlesinger, P. H., Blair, H. C., Beer Stolz, D., Riazanski, V., Ray, E. C., Tourkova, I. L., et al. (2020). Cellular and Extracellular Matrix of Bone, with Principles of Synthesis and Dependency of mineral Deposition on Cell Membrane Transport. Am. J. Physiology-Cell Physiol. 318, C111–C124. Epub 2019 Sep 18. doi:10.1152/ajpcell.00120.2019
Schmidt-Rohlfing, B., Schneider, U., Goost, H., and Silny, J. (2002). Mechanically Induced Electrical Potentials of Articular Cartilage. J. Biomech. 35, 475–482. doi:10.1016/s0021-9290(01)00232-9
Sempertegui, N. D., Narkhede, A. A., Thomas, V., and Rao, S. S. (2018). A Combined Compression Molding, Heating, and Leaching Process for Fabrication of Micro-porous Poly(ε-Caprolactone) Scaffolds. J. Biomater. Sci. Polym. Edition 29, 1978–1993. Epub 2018 Oct 30. doi:10.1080/09205063.2018.1498719
Seo, J.-p., Tanabe, T., Tsuzuki, N., Haneda, S., Yamada, K., Furuoka, H., et al. (2013). Effects of Bilayer Gelatin/β-Tricalcium Phosphate Sponges Loaded with Mesenchymal Stem Cells, Chondrocytes, Bone Morphogenetic Protein-2, and Platelet Rich Plasma on Osteochondral Defects of the Talus in Horses. Res. Vet. Sci. 95, 1210–1216. Epub 2013 Sep 5. doi:10.1016/j.rvsc.2013.08.016
Seong, J. M., Kim, B.-C., Park, J.-H., Kwon, I. K., Mantalaris, A., and Hwang, Y.-S. (2010). Stem Cells in Bone Tissue Engineering. Biomed. Mater. 5, 062001. Epub 2010 Oct 6. doi:10.1088/1748-6041/5/6/062001
Shalumon, K., Sheu, C., Fong, Y., Liao, H.-T., and Chen, J.-P. (2016). Microsphere-Based Hierarchically Juxtapositioned Biphasic Scaffolds Prepared from Poly(Lactic-Co-Glycolic Acid) and Nanohydroxyapatite for Osteochondral Tissue Engineering. Polymers 8, 429. doi:10.3390/polym8120429
Shao, G., Hanaor, D. A. H., Shen, X., and Gurlo, A. (2020). Freeze Casting: From Low‐Dimensional Building Blocks to Aligned Porous Structures-A Review of Novel Materials, Methods, and Applications. Adv. Mater. 32, 1907176. Epub 2020 Mar 12. doi:10.1002/adma.201907176
Sheehy, E. J., Buckley, C. T., and Kelly, D. J. (2012). Oxygen Tension Regulates the Osteogenic, Chondrogenic and Endochondral Phenotype of Bone Marrow Derived Mesenchymal Stem Cells. Biochem. Biophysical Res. Commun. 417, 305–310. Epub 2011 Dec 1. doi:10.1016/j.bbrc.2011.11.105
Sieber, S., Michaelis, M., Gühring, H., Lindemann, S., and Gigout, A. (2020). Importance of Osmolarity and Oxygen Tension for Cartilage Tissue Engineering. BioResearch Open Access 9, 106–115. doi:10.1089/biores.2020.0009
Sing, S. L., Wang, S., Agarwala, S., Wiria, F. E., Ha, T. M. H., and Yeong, W. Y. (2017). Fabrication of Titanium Based Biphasic Scaffold Using Selective Laser Melting and Collagen Immersion. Int. J. Bioprint 3, 007. doi:10.18063/IIB.2017.01.007
Singh, A., Bivalacqua, T. J., and Sopko, N. (2018). Urinary Tissue Engineering: Challenges and Opportunities. Sex. Med. Rev. 6, 35–44. Epub 2017 Oct 21. doi:10.1016/j.sxmr.2017.08.004
Smith, B. D., and Grande, D. A. (2015). The Current State of Scaffolds for Musculoskeletal Regenerative Applications. Nat. Rev. Rheumatol. 11, 213–222. doi:10.1038/nrrheum.2015.27
Sophia Fox, A. J., Bedi, A., and Rodeo, S. A. (2009). The Basic Science of Articular Cartilage: Structure, Composition, and Function. Sports Health 1, 461–468. doi:10.1177/1941738109350438
Sun, Y., Deng, W., Yao, G., Chen, W., Tang, X., Feng, X., et al. (2018). Citrullinated Fibrinogen Impairs Immunomodulatory Function of Bone Marrow Mesenchymal Stem Cells by Triggering Toll-like Receptor. Clin. Immunol. 193, 38–45. Epub 2018 Jan 31. doi:10.1016/j.clim.2018.01.008
Suzuki, A., Minamide, M., Iwaya, C., Ogata, K., and Iwata, J. (2020). Role of Metabolism in Bone Development and Homeostasis. Ijms 21, 8992. doi:10.3390/ijms21238992
Tamjid, E., Bohlouli, M., Mohammadi, S., Alipour, H., and Nikkhah, M. (2020). Sustainable Drug Release from Highly Porous and Architecturally Engineered Composite Scaffolds Prepared by 3D Printing. J. Biomed. Mater. Res. 108, 1426–1438. Epub 2020 Mar 10. doi:10.1002/jbm.a.36914
Tang, D., Tare, R. S., Yang, L.-Y., Williams, D. F., Ou, K.-L., and Oreffo, R. O. C. (2016). Biofabrication of Bone Tissue: Approaches, Challenges and Translation for Bone Regeneration. Biomaterials 83, 363–382. Epub 2016 Jan 9. doi:10.1016/j.biomaterials.2016.01.024
Tourné-Péteilh, C., Robin, B., Lions, M., Martinez, J., Mehdi, A., Subra, G., et al. (2019). Combining Sol-Gel and Microfluidics Processes for the Synthesis of Protein-Containing Hybrid Microgels. Chem. Commun. 55, 13112–13115. doi:10.1039/c9cc04963k
Valot, L., Maumus, M., Montheil, T., Martinez, J., Noël, D., Mehdi, A., et al. (2019). Biocompatible Glycine‐Assisted Catalysis of the Sol‐Gel Process: Development of Cell‐Embedded Hydrogels. Chempluschem 84, 1720–1729. Epub 2019 Oct 24. doi:10.1002/cplu.201900509
Varela-Eirin, M., Loureiro, J., Fonseca, E., Corrochano, S., Caeiro, J. R., Collado, M., et al. (2018). Cartilage Regeneration and Ageing: Targeting Cellular Plasticity in Osteoarthritis. Ageing Res. Rev. 42, 56–71. Epub 2017 Dec 16. doi:10.1016/j.arr.2017.12.006
Vasquez-Sancho, F., Abdollahi, A., Damjanovic, D., and Catalan, G. (2018). Flexoelectricity in Bones. Adv. Mater. 30, 1705316. Epub 2018 Jan 18. doi:10.1002/adma.201705316
Wang, D., Nawabi, D. H., Krych, A. J., Jones, K. J., Nguyen, J., Elbuluk, A. M., et al. (2020). Synthetic Biphasic Scaffolds versus Microfracture for Articular Cartilage Defects of the Knee: A Retrospective Comparative Study. Cartilage. Epub ahead of print. doi:10.1177/1947603520903418
Wang, F., Ying, Z., Duan, X., Tan, H., Yang, B., Guo, L., et al. (2009). Histomorphometric Analysis of Adult Articular Calcified Cartilage Zone. J. Struct. Biol. 168, 359–365. Epub 2009 Aug 31. doi:10.1016/j.jsb.2009.08.010
Wei, W., and Dai, H. (2021). Articular Cartilage and Osteochondral Tissue Engineering Techniques: Recent Advances and Challenges. Bioactive Mater. 6, 4830–4855. doi:10.1016/j.bioactmat.2021.05.011
Wingender, B., Ni, Y., Zhang, Y., Taylor, C., and Gower, L. (2018). Hierarchical Characterization and Nanomechanical Assessment of Biomimetic Scaffolds Mimicking Lamellar Bone via Atomic Force Microscopy Cantilever-Based Nanoindentation. Materials 11, 1257. doi:10.3390/ma11071257
Wu, X., Zhou, M., Jiang, F., Yin, S., Lin, S., Yang, G., et al. (2021). Marginal Sealing Around Integral Bilayer Scaffolds for Repairing Osteochondral Defects Based on Photocurable Silk Hydrogels. Bioactive Mater. 6, 3976–3986. doi:10.1016/j.bioactmat.2021.04.005
Xu, D., Cheng, G., Dai, J., and Li, Z. (2021). Bi-layered Composite Scaffold for Repair of the Osteochondral Defects. Adv. Wound Care 10, 401–414. doi:10.1089/wound.2019.1140
Xuan, H., Hu, H., Geng, C., Song, J., Shen, Y., Lei, D., et al. (2020). Biofunctionalized Chondrogenic Shape-Memory Ternary Scaffolds for Efficient Cell-free Cartilage Regeneration. Acta Biomater. 105, 97–110. Epub 2020 Jan 15. doi:10.1016/j.actbio.2020.01.015
Yao, Q., Nooeaid, P., Detsch, R., Roether, J. A., Dong, Y., Goudouri, O.-M., et al. (2014). Bioglass/chitosan-polycaprolactone Bilayered Composite Scaffolds Intended for Osteochondral Tissue Engineering. J. Biomed. Mater. Res. 102, a–n. Epub 2014 Feb 26. doi:10.1002/jbm.a.35125
Ye, K., Traianedes, K., Robins, S. A., Choong, P. F. M., and Myers, D. E. (2018). Osteochondral Repair Using an Acellular Dermal Matrix-Pilot In Vivo Study in a Rabbit Osteochondral Defect Model. J. Orthop. Res. 36, 1919–1928. Epub 2018 Jan 24. doi:10.1002/jor.23837
Yin, H., Wang, Y., Sun, X., Cui, G., Sun, Z., Chen, P., et al. (2018). Functional Tissue-Engineered Microtissue Derived from Cartilage Extracellular Matrix for Articular Cartilage Regeneration. Acta Biomater. 77, 127–141. Epub 2018 Jul 18. doi:10.1016/j.actbio.2018.07.031
Yunos, D., Ahmad, Z., Salih, V., and Boccaccini, A. (2013). Stratified Scaffolds for Osteochondral Tissue Engineering Applications: Electrospun PDLLA Nanofibre Coated Bioglass-Derived Foams. J. Biomater. Appl. 27, 537–551. doi:10.1177/0885328211414941
Zaborowska, M., Bodin, A., Bäckdahl, H., Popp, J., Goldstein, A., and Gatenholm, P. (2010). Microporous Bacterial Cellulose as a Potential Scaffold for Bone Regeneration. Acta Biomater. 6, 2540–2547. Epub 2010 Jan 11. doi:10.1016/j.actbio.2010.01.004
Zha, K., Li, X., Yang, Z., Tian, G., Sun, Z., Sui, X., et al. (2021). Heterogeneity of Mesenchymal Stem Cells in Cartilage Regeneration: from Characterization to Application. NPJ Regen. Med. 6, 14. doi:10.1038/s41536-021-00122-6
Zhang, B., Huang, J., and Narayan, R. J. (2020). Gradient Scaffolds for Osteochondral Tissue Engineering and Regeneration. J. Mater. Chem. B 8, 8149–8170. doi:10.1039/d0tb00688b
Zhang, J., Liu, H., Ding, J.-X., Wu, J., Zhuang, X.-L., Chen, X.-S., et al. (2016). High-Pressure Compression-Molded Porous Resorbable Polymer/Hydroxyapatite Composite Scaffold for Cranial Bone Regeneration. ACS Biomater. Sci. Eng. 2, 1471–1482. Epub 2016 Aug 10. doi:10.1021/acsbiomaterials.6b00202
Zhang, J., Zhang, X., Hong, Y., Fu, Q., He, Q., Mechakra, A., et al. (2020). Tissue-Adhesive Paint of Silk Microparticles for Articular Surface Cartilage Regeneration. ACS Appl. Mater. Inter. 12, 22467–22478. Epub 2020 May 12. doi:10.1021/acsami.0c01776
Zhang, S., Chen, L., Jiang, Y., Cai, Y., Xu, G., Tong, T., et al. (2013). Bi-layer Collagen/microporous Electrospun Nanofiber Scaffold Improves the Osteochondral Regeneration. Acta Biomater. 9, 7236–7247. Epub 2013 Apr 6. doi:10.1016/j.actbio.2013.04.003
Zhang, S., Chuah, S. J., Lai, R. C., Hui, J. H. P., Lim, S. K., and Toh, W. S. (2018). MSC Exosomes Mediate Cartilage Repair by Enhancing Proliferation, Attenuating Apoptosis and Modulating Immune Reactivity. Biomaterials 156, 16–27. Epub 2017 Nov 21. doi:10.1016/j.biomaterials.2017.11.028
Zhang, Y.-t., Niu, J., Wang, Z., Liu, S., Wu, J., and Yu, B. (2017). Repair of Osteochondral Defects in a Rabbit Model Using Bilayer Poly(Lactide-Co-Glycolide) Scaffolds Loaded with Autologous Platelet-Rich Plasma. Med. Sci. Monit. 23, 5189–5201. doi:10.12659/msm.904082
Zhang, Y., McNeill, E., Tian, H., Soker, S., Andersson, K.-E., Yoo, J. J., et al. (2008). Urine Derived Cells Are a Potential Source for Urological Tissue Reconstruction. J. Urol. 180, 2226–2233. Epub 2008 Sep 20. doi:10.1016/j.juro.2008.07.023
Zhao, X., Shah, D., Gandhi, K., Wei, W., Dwibedi, N., Webster, L., et al. (2019). Clinical, Humanistic, and Economic burden of Osteoarthritis Among Noninstitutionalized Adults in the United States. Osteoarthritis and Cartilage 27, 1618–1626. Epub 2019 Jul 9. doi:10.1016/j.joca.2019.07.002
Zheng, Y.-L., Sun, Y.-P., Zhang, H., Liu, W.-J., Jiang, R., Li, W.-Y., et al. (2015). Mesenchymal Stem Cells Obtained from Synovial Fluid Mesenchymal Stem Cell-Derived Induced Pluripotent Stem Cells on a Matrigel Coating Exhibited Enhanced Proliferation and Differentiation Potential. PLoS One 10, e0144226. doi:10.1371/journal.pone.0144226
Zhou, F., Hong, Y., Zhang, X., Yang, L., Li, J., Jiang, D., et al. (2018). Tough Hydrogel with Enhanced Tissue Integration and In Situ Forming Capability for Osteochondral Defect Repair. Appl. Mater. Today 13, 32–44. doi:10.1016/j.apmt.2018.08.005
Zhou, L., Gjvm, V. O., Malda, J., Stoddart, M. J., Lai, Y., Richards, R. G., et al. (2020). Innovative Tissue‐Engineered Strategies for Osteochondral Defect Repair and Regeneration: Current Progress and Challenges. Adv. Healthc. Mater. 9, 2001008. Epub ahead of print. doi:10.1002/adhm.202001008
Keywords: osteochondral repair, scaffolds, fabrication, tissue engineering, biomaterials
Citation: Fu J-N, Wang X, Yang M, Chen Y-R, Zhang J-Y, Deng R-H, Zhang Z-N, Yu J-K and Yuan F-Z (2022) Scaffold-Based Tissue Engineering Strategies for Osteochondral Repair. Front. Bioeng. Biotechnol. 9:812383. doi: 10.3389/fbioe.2021.812383
Received: 10 November 2021; Accepted: 16 December 2021;
Published: 11 January 2022.
Edited by:
Yin Fang, Nanyang Technological University, SingaporeReviewed by:
Jianxun Ding, Changchun Institute of Applied Chemistry (CAS), ChinaYongliang Ni, Lam Research, United States
Zhen Yang, Nankai University, China
Wenguo Cui, Shanghai Jiao Tong University, China
Copyright © 2022 Fu, Wang, Yang, Chen, Zhang, Deng, Zhang, Yu and Yuan. This is an open-access article distributed under the terms of the Creative Commons Attribution License (CC BY). The use, distribution or reproduction in other forums is permitted, provided the original author(s) and the copyright owner(s) are credited and that the original publication in this journal is cited, in accordance with accepted academic practice. No use, distribution or reproduction is permitted which does not comply with these terms.
*Correspondence: Jia-Kuo Yu, yujiakuo@126.com; Fu-Zhen Yuan, yuanfuzhen2016@163.com
†These authors have contributed equally to this work