Mitochondrial-Dependent and Independent Functions of PINK1
- Guangdong Key Laboratory of Non-human Primate Research, Guangdong-Hongkong-Macau Institute of CNS Regeneration, Jinan University, Guangzhou, China
PINK1 has been characterized as a mitochondrial kinase that can target to damaged mitochondria to initiate mitophagy, a process to remove unhealthy mitochondria for protecting neuronal cells. Mutations of the human PINK1 gene are also found to cause early onset Parkinson’s disease, a neurodegenerative disorder with the pathological feature of mitochondrial dysfunction. Despite compelling evidence from in vitro studies to support the role of PINK1 in regulation of mitochondrial function, there is still lack of strong in vivo evidence to validate PINK1-mediated mitophagy in the brain. In addition, growing evidence indicates that PINK1 also executes function independent of mitochondria. In this review, we discuss the mitochondrial dependent and independent functions of PINK1, aiming at elucidating how PINK1 functions differentially under different circumstances.
Introduction
The PTEN-induced kinase 1 (PINK1) is a serine/threonine kinase whose function has been well characterized by biochemical studies and protein structural analysis (Kane et al., 2014; Kumar et al., 2017; Gan et al., 2022). A large amount of in vitro studies have shown that PINK1 works with Parkin, an E3 ubiquitin ligase, in coordination to target damaged mitochondria for removing unhealthy mitochondria by the lysosome, a process called mitophagy (Matsuda et al., 2010; Kane et al., 2014; Koyano et al., 2014; Ivankovic et al., 2016). In support of the role of PINK1/Parkin in mitochondria, mutations in the PINK1 and Parkin genes are found to cause early onset of Parkinson’s disease that is also associated with mitochondrial dysfunction (Valente et al., 2004; Ishihara-Paul et al., 2008; McLelland et al., 2014; Ham et al., 2020). Identification of the involvement of PINK1/Parkin in mitophagy has expanded the roles of mitochondrial dysfunction and mitophagy in a variety of pathological conditions and diseases. As a result, extensive studies of the function of PINK1/Parkin related to mitochondrial function continue to provide a wealth of information about how PINK1/Parkin govern mitochondria homeostasis.
Despite the prevalent theory that PINK1 is a mitochondrial kinase and its mitochondrial-dependent function plays a critical role in the pathogenesis of PD, there have been unclear and important issues that need to be addressed. First, there is lack of strong in vivo evidence for PINK1/Parkin-mediated mitophagy in animal models. Second, non-mitochondrial dependent function of PINK1 has been reported, but whether this function is related to PD pathogenesis or other pathological conditions remains elusive. In this review, we will discuss the mitochondrial-dependent and non-dependent functions of PINK1, aiming at elucidating how PINK1 functions in vivo and how its dysfunction is involved in PD and other diseases.
Parkinson’s Disease Is Associated With Mitochondrial Defects
Parkinson’s disease (PD) is the second most common neurodegenerative disorder (less prevalent than Alzheimer’s disease) and is characterized by age-dependent and progressive loss of neurons, especially dopamine neurons in the basal ganglia (Tolosa et al., 2006; Bloem et al., 2021). As a result of this selective neurodegeneration, PD is mainly manifested by motor dysfunction, accompanied by cognitive impairment and psychiatric abnormalities (Kalia and Lang, 2015). Pathologically, PD is featured by loss of dopamine neurons in the substantia nigra pars compacta region in the brain and accumulation of a-synuclein positive inclusions (Lewy bodies) (Bloem et al., 2021). The selective neurodegeneration in PD is thought to associate with mitochondrial dysfunction, which is supported by the discovery that mitochondrial toxins, such as 1-methyl-4-phenyl-1,2,3,6-tetrahydropyridine (MPTP), induce selective nigral degeneration in humans and animals (Fornai et al., 2005). Also, postmortem PD patient brain tissue display defects in mitochondrial bioenergetic capacity and function (Schapira et al., 1989; Schapira et al., 1990; Mallach et al., 2019).
Evidence to support the role of PINK1 in mitochondrial homeostasis also includes the association of genetic mutations of the PINK1 gene with PD. Although most of PD patients start to develop symptoms when they reach the age over 50, early onset PD cases were also found in less than 10% of individuals with PD (Golbe, 1991). Most of these early onset cases are caused by genetic mutations, of which the autosomal recessive mutations in the PINK1 gene were identified in early onset PD (Valente et al., 2004; Bonifati et al., 2005; Zhao et al., 2020). Biochemical analysis of PINK1 uncovers its function as a kinase that phosphorylates Parkin. Consistently, recessive mutations in the human Parkin gene were also found to cause early onset PD (Matsumine et al., 1997; Kitada et al., 1998). Furthermore, genetic studies of Drosophila harboring Pink1 mutations demonstrate that mitochondrial pathology caused by loss of Pink1 could be rescued by Parkin (Yang et al., 2006), establishing the theory that PINK1/Parkin act in the same pathway to protect mitochondria. Moreover, PINK1 deficiency in cellular models of PD has been reported to cause a loss of mitochondrial complex I reductive activity (Morais et al., 2014). Loss of functional mitochondrial complex I has been proved to be associated with dopaminergic cell death in PD (Surmeier et al., 2017; González-Rodríguez et al., 2021). However, complex I dysfunction is also implicated in sporadic PD, suggesting that complex I dysfunction can occur independent of PINK1 mutations.
Mitochondrial Dependent Function of PINK1
The first in vivo evidence indicating that PINK1 is involved in regulating mitochondrial quality control came from genetic studies in Drosophila, which revealed that PINK1 null mutant flies showed apoptotic muscle degeneration, mitochondrial defects, and male sterility (Clark et al., 2006). Subsequently, a large body of biochemical studies have proved that PINK1 functions as a mitochondrial kinase. PINK1 is a 581 amino acid protein consisting of an N-terminal mitochondrial targeting motif that contains a transmembrane domain (110 amino acids long), a highly conserved kinase domain with three insertions in the N lobe, and a C-terminal autoregulatory sequence (Beilina et al., 2005; Cardona et al., 2011; Kumar et al., 2017). Mounting evidence from biochemical and in vitro studies indicates that PINK1 and Parkin work together in the same signaling pathway, as both proteins target damaged mitochondria to the lysosomes for clearance of the unhealthy mitochondria, a process called mitophagy (Pickrell and Youle, 2015; Ivankovic et al., 2016; Nguyen et al., 2016). The most compelling evidence to support the role of PINK1/Parkin in mitophagy is that PINK1 is targeted to mitochondria when cultured cells are under mitochondrial stress induced by the mitochondria-depolarizing agent such as CCCP (carbonyl cyanide m-chlorophenylhydrazine). Once PINK1 is localized on mitochondria, it phosphorylates Parkin and ubiquitin to recruit them to the damaged mitochondria, leading to the ubiquitination of mitochondrial proteins. Then the autophagic adaptor proteins such as p62/SQSTM1/sequestosome-1 were recruited to the damaged mitochondria and mediate the removal of damaged mitochondria by lysosomes (Nguyen et al., 2016). During this process, PINK1 acts as a key sensor of mitochondrial damage whereas Parkin amplifies this damage signal by facilitating the formation of ubiquitin chains, which recruit more Parkin to the damaged mitochondria (Harper et al., 2018). Further, analysis of the crystal structure of insect PINK1 bound to ubiquitin provides a structural base for the interactions of PINK1 with Parkin and ubiquitin (Kumar et al., 2017; Schubert et al., 2017; Gan et al., 2022). All these findings generate important insights into the function of PINK1 and strongly support the theory that PINK1 functions as a mitochondrial kinase and plays a pivotal role in mitophagy, an important intracellular process that is involved in a variety of cellular functions. Consistently, several lines of evidence indicate that PINK1 confers protection against mitochondria dependent apoptosis induced by both intrinsic stress and environmental insults (Gautier et al., 2008; Wang et al., 2011; Huang et al., 2017).
Investigation of the function of PINK1 for mitophagy also yield important finding that endogenous PINK1 is synthesized constitutively in the cytosol as a full-length precursor (∼63–68 kDa). Upon import into mitochondria, PINK1 is proteolytically cleaved to produce its mature form (∼52–55 kDa) that is subsequently re-translocated to the cytosol, resulting in a rapid turnover and low steady state levels (Liu et al., 2017). Indeed, N-terminal region of PINK1, which is responsible for targeting PINK1 to the mitochondria, contains proteolytic sites that can be cleaved by MPP (mitochondrial processing peptidase) and PARL (presenilin-associated rhomboid-like protease) successively (Jin et al., 2010; Deas et al., 2011). These cleavages release PINK1 from mitochondria to produce a cytosolic form containing the kinase domain (Figure 1). However, when mitochondria are damaged, PINK1 is stabilized on the mitochondrial membrane, which can also be activated by kinetin that can amplify the catalytic activity of PINK1 (Hertz et al., 2013), to phosphorylate Parkin and ubiquitin to initiate mitophagy (Kane et al., 2014). All these findings convincingly demonstrate the mitochondrial-dependent function of PINK1 in cells. Since PINK1-mediated mitophagy is dependent on its targeting to mitochondria whereas cleavage of PINK1 via proteases can dissociate PINK1 from mitochondria and therefore inhibit PINK1’s function on mitophagy, suppressing proteases activity to cleave PINK1 is presumably able to enhance PINK1-dependent mitophagy process. In this regard, small-molecule inhibitors of the mitochondrial protease PARL that can cleave PINK1 could potentially enhance the activity of PINK1-depedendent mitophagy (Parsons et al., 2021).
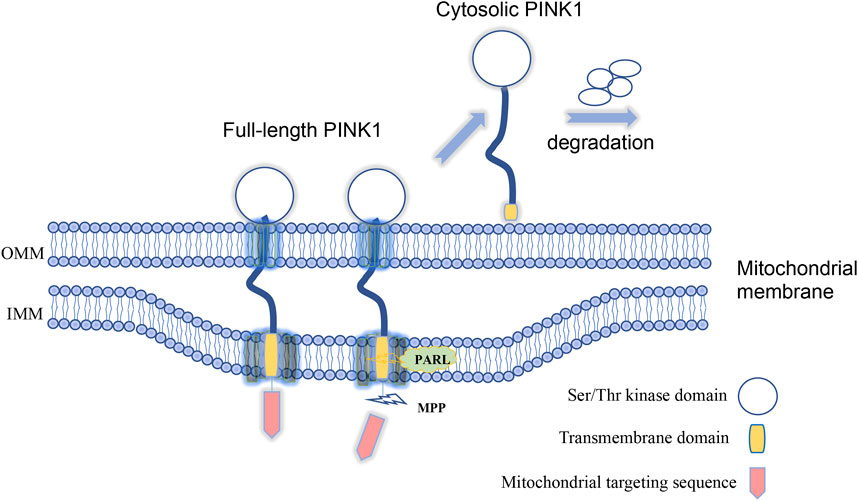
FIGURE 1. Full-length PINK1 is able to target to mitochondria and is cleaved by proteases to generate cytosolic form of PINK1 upon mitochondria damage. Lack of in vivo evidence for PINK1-mediated mitophagy.
Lack of in vivo evidence for PINK1-mediated mitophagy
However, most of studies of PINK1 used cultured cells or in vitro systems to investigate mitochondrial-dependent function of full-length PINK1 in the field. Several groups have established PINK1 KO mouse models, aiming at identifying phenotypes associated with loss of PINK1 (Table 1). However, all these rodent KO models do not recapitulate the neurodegeneration seen in PD patient brains (Kitada et al., 2007; Akundi et al., 2011; Ge et al., 2020). Although rat PINK1 KO model was initially report to show DA neuronal degeneration (Dave et al., 2014), this phenotype is not severe as that in PD patient brain and was unable to be confirmed later by a different group (de Haas et al., 2019). Although PINK1/Parkin knockout mouse models are unable to show typical PD phenotypes, Parkin knockout could increase the vulnerability of dopaminergic neurons to exhaustive exercise via STING, a central regulator of the type I interferon response to cytosolic DNA (Sliter et al., 2018), suggesting that loss of Parkin alone is not sufficient to induce neurodegeneration in mice. To rigorously investigate the in vivo function of PINK1 for mitophagy, a knock-in mouse model, in which the codon encoding Parkin Ser65 was mutated to Ala65 to prevent its phosphorylation by PINK1, was established but this model still showed no clear neurodegeneration or nigrostriatal mitophagy impairment (McWilliams et al., 2018a).
Another strong evidence indicating that loss of PINK1 does not impact mitochondria homeostasis is the lack of influence of PINK1 on the basal mitophagy activity in Drosophila and mice (McWilliams et al., 2018b; Lee et al., 2018). In the PINK1 null fly and mice, in vivo mitophagy assay, which was performed using mito-QC or mt-Keima as a mitophagy reporter, did not show alteration as compared with wild type animals. Although the complex I subunit NDUFA10 was found to be phosphorylated by PINK1, transgenic overexpression of NDUFA10 can rescue Drosophila pink1 mutants independent of mitophagy (Pogson et al., 2014). Apart from these findings, mass spectrometry analysis of PINK1 knockout rodent did not show significant alterations in the expression levels of mitochondrial proteins (Stauch et al., 2016). As for mitochondrial function studies, inconsistent or mild alterations, at least not striking as in vitro findings, were found among the PINK1 KO animal models (Zhuang et al., 2016; Yamada et al., 2019). Also, PINK1/Parkin axis is not only the signaling pathway to regulate mitophagy, as growing evidence indicates the presence of PINK1/Parkin-independent mitophagy under in vivo or physiological conditions (Munson et al., 2021; Munson et al., 2022; Terešak et al., 2022). All these raise an important issue of whether PINK1 acts differentially in vitro and in vivo to mediate mitochondrial-dependent and independent functions.
Our recent studies using non-human primate model demonstrate for the first time that loss of PINK1 in the mammalian brain can cause neuronal loss (Table 1). We found that the deletion of the large PINK1 DNA fragment by CRISPR/Cas9 can induce neuronal loss in the developing and adult monkey brains (Yang et al., 2019a; Yang et al., 2022). The homozygous deletion of a large region of the PINK1 gene has not been found in humans, perhaps because such deletion is embryonic lethal in humans. However, CRISPR/Cas9-mediated deletion of the monkey PINK1 gene can completely eliminate the expression of PINK1 to elicit severe neurodegeneration in the non-human primate brain (Yang et al., 2019a), which also suggests that PINK1 point mutations found in patients with PD may partially impair PINK1 function to cause age-dependent neurodegeneration. One of the important findings from the non-human primate models is the striking neuronal loss without significant impact on mitochondria homeostasis (Yang et al., 2022). Also, the severe neuronal loss in the monkey brain is in clear contrast to the absence of neurodegeneration in mouse models that have completely deleted the Pink1 gene, suggesting that PINK1’s function is species-dependent.
Mitochondria Independent Function of PINK1
Although PINK1 is known to be cleaved to a truncated form by removing its N-terminal mitochondrial targeting domain, the functions of this cytosolic form of PINK1 have not been well characterized, and most of investigation focuses on the mitochondrial-dependent function of full-length PINK1 in the field. However, emerging evidence indicates that cytosolic PINK1 functions in many aspects to regulate cellular functions. In addition to PINK1-mediated phosphorylation of Parkin and ubiquitin (Eiyama and Okamoto, 2015), growing evidence indicates that the kinase activity of PINK1 spans to other substrates, including Drp1 (Han et al., 2020), TRAP1 (Pridgeon et al., 2007), Mfn2 (Chen et al., 2013), Miro (Wang et al., 2011), Bcl-xL (Arena et al., 2013), complex I subunit NdufA10 (Morais et al., 2014), and HtrA2 (Plun-Favreau et al., 2007). Phosphorylation of these signaling molecules appears to be independent of mitochondria but important for cell survival. In line with this idea, cytosolic PINK1 can mediate neuroprotection, since PINK1 lacking the mitochondrial targeting sequences, which can be produced by proteolytic process via N-end rule pathway, protects against MPTP-induced toxicity in mice (Haque et al., 2008). In addition, cytosolic PINK1 cannot promote mitophagy (Geisler et al., 2010; Narendra et al., 2010), suggesting that the pro-survival activity of PINK1 is not related to the mitophagy-inducing activity.
Investigation of PINK1-targeted monkey models also strongly supports the notion that PINK1 functions as a kinase in vivo, at least in the primate brains. Loss of PINK1 leads to marked decrease in phosphorylation of proteins that are important for neuronal survival (Yang et al., 2022). In support of this finding, the major form of PINK1 seen in the primate brain is the cytosolic form (55 kD) that lacks N-terminal region (Yang et al., 2022). This form of PINK1 contains the intact kinase domain and is presumably able to phosphorylate proteins in a mitochondrial independent manner. Earlier studies have revealed that cytosolic PINK1 promotes neuronal plasticity and differentiation, as PINK1-deficient cortical and midbrain neurons display defective dendritic morphology, and overexpression of truncated and cytosolic PINK1 could rescue this phenotype and also induce neuronal differentiation in SH-SH5Y neuronal cells (Dagda et al., 2014). The role of PINK1 in regulating neuronal differentiation is also supported by the finding from zebrafish and human organoid models that PINK1 deficiency impedes dopaminergic neuron neurogenesis (Brown et al., 2021).
PINK1’s function seems to be not restricted to the brain, as PINK1 is also upregulated in breast, colorectal, and endometrial cancer tissues, whereas PINK1 inhibition reduces cancer cell proliferation (Zhang et al., 2017). The cytosolic PINK1 has been implicated in signaling cascades critical to cell growth and survival, including the PI3-kinase (PI3K)/Akt, valosin-containing protein (VCP), and protein kinase A (PKA) pathways (Akundi et al., 2012; Soutar et al., 2018; Wang et al., 2018; Boonying et al., 2019). PINK1 deficiency in Drosophila is found to cause multiple growth defects independent of Parkin (Han et al., 2021). Along with the mitochondrial function of PINK1, the role of PINK1 in regulating cell cycle has also been reported, which is more likely to relate to tumor and cancer cells in which PINK1 expression is noticeably altered (O'Flanagan et al., 2015; Leites and Morais, 2018). PINK1 was initially identified as a downstream effector of phosphatase and tensin homolog (PTEN), a tumor suppressor that is frequently mutated in various types of human cancers (Unoki and Nakamura, 2001). Thus, more studies are required to investigate the mitochondrial-independent function of PINK1 and the relevance of this function to physiological and pathological scenarios.
Questions That Need to Be Addressed
The above description of mitochondrial-dependent and independent functions of PINK1 clearly indicates that PINK1 functions differently under different circumstances (Figure 2). It is difficult to reconcile the well-characterized mitophagy function of PINK1 and the absence of in vivo evidence for PINK1-mediated mitophagy in some animal models. It should be noted that most of in vitro studies of mitochondrial-dependent function of PINK1 involve overexpression of PINK1 or exogenously transfected PINK1 in combination with a mitochondrial depolarizing agent. It has been well recognized in the field that endogenous PINK1 is unstable and is very difficult to be detected in the rodent animals. This would explain that the intrinsically very low level of endogenous PINK1 is unable to recapitulate the in vitro function that is induced by overexpressed PINK1 with acute or extraordinary stress on mitochondria. On the other hand, endogenous PINK1 is more abundant in the primate brain and mainly functions as a kinase to phosphorylate neuronal proteins for maintaining the survival of primate neuronal cells. Thus, expression levels of PINK1 in vivo and in vitro are highly likely to account for different functions of PINK1. Also, as full-length PINK1 is more likely to act as a mitochondrial kinase whereas its cleaved products exist in the cytosol to function independent of mitochondria, the proteolytic processing of PINK1 determines the specific function of different PINK1 forms.
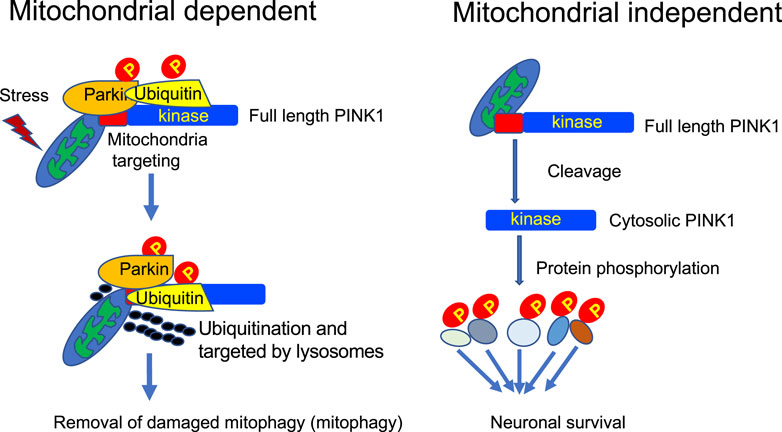
FIGURE 2. Mitochondrial-dependent and independent functions of PINK1. In the primate brain, PINK1 can phosphorylate a large number of proteins, including those for regulating mitochondrial function, to maintain neuronal survival.
PINK1 seems to be a multifaceted protein acting at the crossroads of various pathways critical for cell survival, mitochondria quality control, and cell cycle regulation. The various functions of PINK1 raise many important issues that need to be well addressed and also pose challenges to our understanding of how these functions are regulated. First, why is there species-dependent expression of PINK1, for example, why is PINK1 undetectable in mice but abundant in the primates? The regulation of PINK1 expression is more likely mediated at translational and/or protein stability level, as PINK1 mRNA is ubiquitously and abundantly expressed across different species (Blackinton et al., 2007). However, rigorous investigation of mechanisms underlying PINK1 protein expression and cleavage in vivo remains to be conducted. Lack of detectable expression of PINK1 in the rodent models makes it difficult to use small mammals to explore this important issue. Large animal models have been found to provide new insights into the pathogenesis of neurodegenerative diseases (Sun et al., 2022; Yin et al., 2022). However, the absent phenotypes of pig models that have deleted the PINK1 gene (Zhou et al., 2015; Wang et al., 2016) suggest that endogenous PINK1 may also be expressed at a low level in the swine species. Thus, identification of the abundant expression of PINK1 kinase in the primate brains and the phenotypes due to PINK1 deficiency in the monkey model indicates that the non-human primates would be a suitable animal model for delineating the in vivo regulation of PINK1 expression and function.
Second, to what extent dose PINK1 mitochondrial-dependent function play a role in vivo, especially under pathological conditions? Mitophagy has been well documented and characterized for its protection against cell death. There is no doubt that PINK1/Parkin mediates mitophagy in cultured cells, but an important and outstanding question is whether this occurs in vivo when PINK1/Parkin are expressed at the endogenous level and under physiological conditions. Is full-length PINK1, which is able to localize to mitochondria, or cytosolic PINK1, which loses the ability to associate with mitochondria, more important for cell survival and function, and how do these different forms of PINK1 coordinate their functions in vivo?
Lastly, how important is PINK1’s function beyond mitochondria? Cytosolic PINK1 is evidently able to function as a kinase independent of mitochondria. Most kinases are key regulators of multiple cellular processes, and their functions and expressions are regulated by complex and sophisticated mechanisms whereas dysregulation of their expression can lead to diseases (Burke, 2018). If PINK1’s kinase function is critical for neuronal survival and differentiation, can improving its catalytic activity reduce neurodegeneration that is resulted from loss of PINK1 function? On the other hand, because PINK1 is upregulated in many tumor cells, can inhibition of its kinase activity suppress abnormal cell proliferation to treat tumorigenesis? Continued study of PINK1 beyond its mitochondrial functions may potentially shed light on novel therapeutic approaches.
Author Contributions
X-JL, WY, and XC wrote the manuscript. QW and SL edited the manuscript. All discussed and approved the overall structure of the review.
Funding
This work was supported by The National Natural Science Foundation of China (32070534, 81830032, 31872779, 82071421, and 81873736); Guangzhou Key Research Program on Brain Science (202007030008), Department of Science and Technology of Guangdong Province (2018B030337001, 2021ZT09Y007; 2020B121201006).
Conflict of Interest
The authors declare that the research was conducted in the absence of any commercial or financial relationships that could be construed as a potential conflict of interest.
Publisher’s Note
All claims expressed in this article are solely those of the authors and do not necessarily represent those of their affiliated organizations, or those of the publisher, the editors and the reviewers. Any product that may be evaluated in this article, or claim that may be made by its manufacturer, is not guaranteed or endorsed by the publisher.
References
Akundi, R. S., Huang, Z., Eason, J., Pandya, J. D., Zhi, L., Cass, W. A., et al. (2011). Increased Mitochondrial Calcium Sensitivity and Abnormal Expression of Innate Immunity Genes Precede Dopaminergic Defects in Pink1-Deficient Mice. PLoS One 6 (1), e16038. doi:10.1371/journal.pone.0016038
Akundi, R. S., Zhi, L., and Büeler, H. (2012). PINK1 Enhances Insulin-like Growth Factor-1-dependent Akt Signaling and Protection against Apoptosis. Neurobiol. Dis. 45 (1), 469–478. doi:10.1016/j.nbd.2011.08.034
Arena, G., Gelmetti, V., Torosantucci, L., Vignone, D., Lamorte, G., De Rosa, P., et al. (2013). PINK1 Protects against Cell Death Induced by Mitochondrial Depolarization, by Phosphorylating Bcl-xL and Impairing its Pro-apoptotic Cleavage. Cell. Death Differ. 20 (7), 920–930. doi:10.1038/cdd.2013.19
Beilina, A., Van Der Brug, M., Ahmad, R., Kesavapany, S., Miller, D. W., Petsko, G. A., et al. (2005). Mutations in PTEN-Induced Putative Kinase 1 Associated with Recessive Parkinsonism Have Differential Effects on Protein Stability. Proc. Natl. Acad. Sci. U.S.A. 102 (16), 5703–5708. doi:10.1073/pnas.0500617102
Blackinton, J. G., Anvret, A., Beilina, A., Olson, L., Cookson, M. R., and Galter, D. (2007). Expression of PINK1 mRNA in Human and Rodent Brain and in Parkinson's Disease. Brain Res. 1184, 10–16. doi:10.1016/j.brainres.2007.09.056
Bloem, B. R., Okun, M. S., and Klein, C. (2021). Parkinson's Disease. Lancet 397 (10291), 2284–2303. doi:10.1016/s0140-6736(21)00218-x
Bonifati, V., Rohe, C. F., Breedveld, G. J., Fabrizio, E., De Mari, M., Tassorelli, C., et al. (2005). Early-onset Parkinsonism Associated with PINK1 Mutations: Frequency, Genotypes, and Phenotypes. Neurology 65 (1), 87–95. doi:10.1212/01.wnl.0000167546.39375.82
Boonying, W., Joselin, A., Huang, E., Qu, D., Safarpour, F., Iyirhiaro, G. O., et al. (2019). Pink1 regulatesFKBP5 Interaction withAKT/PHLPPand Protects Neurons from Neurotoxin Stress Induced byMPP+. J. Neurochem. 150 (3), 312–329. doi:10.1111/jnc.14683
Brown, S. J., Boussaad, I., Jarazo, J., Fitzgerald, J. C., Antony, P., Keatinge, M., et al. (2021). PINK1 Deficiency Impairs Adult Neurogenesis of Dopaminergic Neurons. Sci. Rep. 11 (1), 6617. doi:10.1038/s41598-021-84278-7
Burke, J. E. (2018). Structural Basis for Regulation of Phosphoinositide Kinases and Their Involvement in Human Disease. Mol. Cell. 71 (5), 653–673. doi:10.1016/j.molcel.2018.08.005
Cardona, F., Sánchez‐Mut, J. V., Dopazo, H., and Pérez‐Tur, J. (2011). Phylogenetic and In Silico Structural Analysis of the Parkinson Disease‐related Kinase PINK1. Hum. Mutat. 32 (4), 369–378. doi:10.1002/humu.21444
Chen, Y., Dorn, G. W., and 2nd, (2013). PINK1-phosphorylated Mitofusin 2 Is a Parkin Receptor for Culling Damaged Mitochondria. Science 340 (6131), 471–475. doi:10.1126/science.1231031
Clark, I. E., Dodson, M. W., Jiang, C., Cao, J. H., Huh, J. R., Seol, J. H., et al. (2006). Drosophila Pink1 Is Required for Mitochondrial Function and Interacts Genetically with Parkin. Nature 441 (7097), 1162–1166. doi:10.1038/nature04779
Dagda, R. K., Pien, I., Wang, R., Zhu, J., Wang, K. Z. Q., Callio, J., et al. (2014). Beyond the Mitochondrion: Cytosolic PINK1 Remodels Dendrites through Protein Kinase A. J. Neurochem. 128 (6), 864–877. doi:10.1111/jnc.12494
Dave, K. D., De Silva, S., Sheth, N. P., Ramboz, S., Beck, M. J., Quang, C., et al. (2014). Phenotypic Characterization of Recessive Gene Knockout Rat Models of Parkinson's Disease. Neurobiol. Dis. 70, 190–203. doi:10.1016/j.nbd.2014.06.009
de Haas, R., Heltzel, L. C. M. W., Tax, D., van den Broek, P., Steenbreker, H., Verheij, M. M. M., et al. (2019). To Be or Not to Be Pink(1): Contradictory Findings in an Animal Model for Parkinson's Disease. Brain Commun. 1 (1), fcz016. doi:10.1093/braincomms/fcz016
Deas, E., Plun-Favreau, H., Gandhi, S., Desmond, H., Kjaer, S., Loh, S. H. Y., et al. (2011). PINK1 Cleavage at Position A103 by the Mitochondrial Protease PARL. Hum. Mol. Genet. 20 (5), 867–879. doi:10.1093/hmg/ddq526
Eiyama, A., and Okamoto, K. (2015). PINK1/Parkin-mediated Mitophagy in Mammalian Cells. Curr. Opin. Cell. Biol. 33, 95–101. doi:10.1016/j.ceb.2015.01.002
Fornai, F., Schlüter, O. M., Lenzi, P., Gesi, M., Ruffoli, R., Ferrucci, M., et al. (2005). Parkinson-like Syndrome Induced by Continuous MPTP Infusion: Convergent Roles of the Ubiquitin-Proteasome System and α-synuclein. Proc. Natl. Acad. Sci. U.S.A. 102 (9), 3413–3418. doi:10.1073/pnas.0409713102
Gan, Z. Y., Callegari, S., Cobbold, S. A., Cotton, T. R., Mlodzianoski, M. J., Schubert, A. F., et al. (2022). Activation Mechanism of PINK1. Nature 602 (7896), 328–335. doi:10.1038/s41586-021-04340-2
Gautier, C. A., Kitada, T., and Shen, J. (2008). Loss of PINK1 Causes Mitochondrial Functional Defects and Increased Sensitivity to Oxidative Stress. Proc. Natl. Acad. Sci. U.S.A. 105 (32), 11364–11369. doi:10.1073/pnas.0802076105
Ge, P., Dawson, V. L., and Dawson, T. M. (2020). PINK1 and Parkin Mitochondrial Quality Control: a Source of Regional Vulnerability in Parkinson's Disease. Mol. Neurodegener. 15 (1), 20. doi:10.1186/s13024-020-00367-7
Geisler, S., Holmström, K. M., Skujat, D., Fiesel, F. C., Rothfuss, O. C., Kahle, P. J., et al. (2010). PINK1/Parkin-mediated Mitophagy Is Dependent on VDAC1 and p62/SQSTM1. Nat. Cell. Biol. 12 (2), 119–131. doi:10.1038/ncb2012
Golbe, L. I. (1991). Young‐onset Parkinson's Disease. Neurology 41 (2Pt 1), 168. doi:10.1212/wnl.41.2_part_1.168
González-Rodríguez, P., Zampese, E., Stout, K. A., Guzman, J. N., Ilijic, E., Yang, B., et al. (2021). Disruption of Mitochondrial Complex I Induces Progressive Parkinsonism. Nature 599 (7886), 650–656. doi:10.1038/s41586-021-04059-0
Ham, S. J., Lee, D., Yoo, H., Jun, K., Shin, H., and Chung, J. (2020). Decision between Mitophagy and Apoptosis by Parkin via VDAC1 Ubiquitination. Proc. Natl. Acad. Sci. U.S.A. 117 (8), 4281–4291. doi:10.1073/pnas.1909814117
Han, H., Tan, J., Wang, R., Wan, H., He, Y., Yan, X., et al. (2020). PINK 1 Phosphorylates Drp1 S616 to Regulate Mitophagy‐independent Mitochondrial Dynamics. EMBO Rep. 21 (8), e48686. doi:10.15252/embr.201948686
Han, Y., Zhuang, N., and Wang, T. (2021). Roles of PINK1 in Regulation of Systemic Growth Inhibition Induced by Mutations of PTEN in Drosophila. Cell. Rep. 34 (12), 108875. doi:10.1016/j.celrep.2021.108875
Haque, M. E., Thomas, K. J., D'Souza, C., Callaghan, S., Kitada, T., Slack, R. S., et al. (2008). Cytoplasmic Pink1 Activity Protects Neurons from Dopaminergic Neurotoxin MPTP. Proc. Natl. Acad. Sci. U.S.A. 105 (5), 1716–1721. doi:10.1073/pnas.0705363105
Harper, J. W., Ordureau, A., and Heo, J.-M. (2018). Building and Decoding Ubiquitin Chains for Mitophagy. Nat. Rev. Mol. Cell. Biol. 19 (2), 93–108. doi:10.1038/nrm.2017.129
Hertz, N. T., Berthet, A., Sos, M. L., Thorn, K. S., Burlingame, A. L., Nakamura, K., et al. (2013). A Neo-Substrate that Amplifies Catalytic Activity of Parkinson's-Disease-Related Kinase PINK1. Cell. 154 (4), 737–747. doi:10.1016/j.cell.2013.07.030
Huang, E., Qu, D., Huang, T., Rizzi, N., Boonying, W., Krolak, D., et al. (2017). PINK1-mediated Phosphorylation of LETM1 Regulates Mitochondrial Calcium Transport and Protects Neurons against Mitochondrial Stress. Nat. Commun. 8 (1), 1399. doi:10.1038/s41467-017-01435-1
Ishihara-Paul, L., Hulihan, M. M., Kachergus, J., Upmanyu, R., Warren, L., Amouri, R., et al. (2008). PINK1 Mutations and Parkinsonism. Neurology 71 (12), 896–902. doi:10.1212/01.wnl.0000323812.40708.1f
Ivankovic, D., Chau, K. Y., Schapira, A. H. V., and Gegg, M. E. (2016). Mitochondrial and Lysosomal Biogenesis Are Activated Following PINK 1/parkin‐mediated Mitophagy. J. Neurochem. 136 (2), 388–402. doi:10.1111/jnc.13412
Jin, S. M., Lazarou, M., Wang, C., Kane, L. A., Narendra, D. P., and Youle, R. J. (2010). Mitochondrial Membrane Potential Regulates PINK1 Import and Proteolytic Destabilization by PARL. J. Cell. Biol. 191 (5), 933–942. doi:10.1083/jcb.201008084
Kalia, L. V., and Lang, A. E. (2015). Parkinson's Disease. Lancet 386 (9996), 896–912. doi:10.1016/s0140-6736(14)61393-3
Kane, L. A., Lazarou, M., Fogel, A. I., Li, Y., Yamano, K., Sarraf, S. A., et al. (2014). PINK1 Phosphorylates Ubiquitin to Activate Parkin E3 Ubiquitin Ligase Activity. J. Cell. Biol. 205 (2), 143–153. doi:10.1083/jcb.201402104
Kitada, T., Asakawa, S., Hattori, N., Matsumine, H., Yamamura, Y., Minoshima, S., et al. (1998). Mutations in the Parkin Gene Cause Autosomal Recessive Juvenile Parkinsonism. Nature 392 (6676), 605–608. doi:10.1038/33416
Kitada, T., Pisani, A., Porter, D. R., Yamaguchi, H., Tscherter, A., Martella, G., et al. (2007). Impaired Dopamine Release and Synaptic Plasticity in the Striatum of PINK1 -deficient Mice. Proc. Natl. Acad. Sci. U.S.A. 104 (27), 11441–11446. doi:10.1073/pnas.0702717104
Koyano, F., Okatsu, K., Kosako, H., Tamura, Y., Go, E., Kimura, M., et al. (2014). Ubiquitin Is Phosphorylated by PINK1 to Activate Parkin. Nature 510 (7503), 162–166. doi:10.1038/nature13392
Kumar, A., Tamjar, J., Waddell, A. D., Woodroof, H. I., Raimi, O. G., Shaw, A. M., et al. (2017). Structure of PINK1 and Mechanisms of Parkinson's Disease-Associated Mutations. Elife 6. doi:10.7554/eLife.29985
Lee, J. J., Sanchez-Martinez, A., Zarate, A. M., Benincá, C., Mayor, U., Clague, M. J., et al. (2018). Basal Mitophagy Is Widespread in Drosophila but Minimally Affected by Loss of Pink1 or Parkin. J. Cell. Biol. 217 (5), 1613–1622. doi:10.1083/jcb.201801044
Leites, E. P., and Morais, V. A. (2018). Mitochondrial Quality Control Pathways: PINK1 Acts as a Gatekeeper. Biochem. Biophysical Res. Commun. 500 (1), 45–50. doi:10.1016/j.bbrc.2017.06.096
Li, H., Wu, S., Ma, X., Li, X., Cheng, T., Chen, Z., et al. (2021). Co-editing PINK1 and DJ-1 Genes via Adeno-Associated Virus-Delivered CRISPR/Cas9 System in Adult Monkey Brain Elicits Classical Parkinsonian Phenotype. Neurosci. Bull. 37 (9), 1271–1288. doi:10.1007/s12264-021-00732-6
Liu, Y., Guardia-Laguarta, C., Yin, J., Erdjument-Bromage, H., Martin, B., James, M., et al. (2017). The Ubiquitination of PINK1 Is Restricted to its Mature 52-kDa Form. Cell. Rep. 20 (1), 30–39. doi:10.1016/j.celrep.2017.06.022
Mallach, A., Weinert, M., Arthur, J., Gveric, D., Tierney, T. S., and Alavian, K. N. (2019). Post Mortem Examination of Parkinson's Disease Brains Suggests Decline in Mitochondrial Biomass, Reversed by Deep Brain Stimulation of Subthalamic Nucleus. FASEB J. 33 (6), 6957–6961. doi:10.1096/fj.201802628R
Matsuda, N., Sato, S., Shiba, K., Okatsu, K., Saisho, K., Gautier, C. A., et al. (2010). PINK1 Stabilized by Mitochondrial Depolarization Recruits Parkin to Damaged Mitochondria and Activates Latent Parkin for Mitophagy. J. Cell. Biol. 189 (2), 211–221. doi:10.1083/jcb.200910140
Matsumine, H., Saito, M., Shimoda-Matsubayashi, S., Tanaka, H., Ishikawa, A., Nakagawa-Hattori, Y., et al. (1997). Localization of a Gene for an Autosomal Recessive Form of Juvenile Parkinsonism to Chromosome 6q25.2-27. Am. J. Hum. Genet. 60 (3), 588–596.
McLelland, G.-L., Soubannier, V., Chen, C. X., McBride, H. M., and Fon, E. A. (2014). Parkin and PINK1 Function in a Vesicular Trafficking Pathway Regulating Mitochondrial Quality Control. Embo J. 33 (4), a–n. doi:10.1002/embj.201385902
McWilliams, T. G., Barini, E., Pohjolan-Pirhonen, R., Brooks, S. P., Singh, F., Burel, S., et al. (2018a). Phosphorylation of Parkin at Serine 65 Is Essential for its Activation In Vivo. Open Biol. 8 (11), 180108. doi:10.1098/rsob.180108
McWilliams, T. G., Prescott, A. R., Montava-Garriga, L., Ball, G., Singh, F., Barini, E., et al. (2018b). Basal Mitophagy Occurs Independently of PINK1 in Mouse Tissues of High Metabolic Demand. Cell. Metab. 27 (2), 439–449. e435. doi:10.1016/j.cmet.2017.12.008
Morais, V. A., Haddad, D., Craessaerts, K., De Bock, P.-J., Swerts, J., Vilain, S., et al. (2014). PINK1 Loss-Of-Function Mutations Affect Mitochondrial Complex I Activity via NdufA10 Ubiquinone Uncoupling. Science 344 (6180), 203–207. doi:10.1126/science.1249161
Munson, M. J., Mathai, B. J., Ng, M. Y. W., Trachsel-Moncho, L., de la Ballina, L. R., Schultz, S. W., et al. (2021). GAK and PRKCD Are Positive Regulators of PRKN-independent Mitophagy. Nat. Commun. 12 (1), 6101. doi:10.1038/s41467-021-26331-7
Munson, M. J., Mathai, B. J., Ng, M. Y. W., Trachsel-Moncho, L., de la Ballina, L. R., and Simonsen, A. (2022). GAK and PRKCD Kinases Regulate Basal Mitophagy. Autophagy 18 (2), 467–469. doi:10.1080/15548627.2021.2015154
Narendra, D. P., Jin, S. M., Tanaka, A., Suen, D.-F., Gautier, C. A., Shen, J., et al. (2010). PINK1 Is Selectively Stabilized on Impaired Mitochondria to Activate Parkin. PLoS Biol. 8 (1), e1000298. doi:10.1371/journal.pbio.1000298
Nguyen, T. N., Padman, B. S., and Lazarou, M. (2016). Deciphering the Molecular Signals of PINK1/Parkin Mitophagy. Trends Cell. Biol. 26 (10), 733–744. doi:10.1016/j.tcb.2016.05.008
O’Flanagan, C. H., Bowers, L. W., and Hursting, S. D. (2015). A Weighty Problem: Metabolic Perturbations and the Obesity-Cancer Link. Horm. Mol. Biol. Clin. Investig. 23 (2), 47–57. doi:10.1515/hmbci-2015-0022
Parsons, W. H., Rutland, N. T., Crainic, J. A., Cardozo, J. M., Chow, A. S., Andrews, C. L., et al. (2021). Development of Succinimide-Based Inhibitors for the Mitochondrial Rhomboid Protease PARL. Bioorg. Med. Chem. Lett. 49, 128290. doi:10.1016/j.bmcl.2021.128290
Pickrell, A. M., and Youle, R. J. (2015). The Roles of PINK1, Parkin, and Mitochondrial Fidelity in Parkinson's Disease. Neuron 85 (2), 257–273. doi:10.1016/j.neuron.2014.12.007
Plun-Favreau, H., Klupsch, K., Moisoi, N., Gandhi, S., Kjaer, S., Frith, D., et al. (2007). The Mitochondrial Protease HtrA2 Is Regulated by Parkinson's Disease-Associated Kinase PINK1. Nat. Cell. Biol. 9 (11), 1243–1252. doi:10.1038/ncb1644
Pogson, J. H., Ivatt, R. M., Sanchez-Martinez, A., Tufi, R., Wilson, E., Mortiboys, H., et al. (2014). The Complex I Subunit NDUFA10 Selectively Rescues Drosophila Pink1 Mutants through a Mechanism Independent of Mitophagy. PLoS Genet. 10 (11), e1004815. doi:10.1371/journal.pgen.1004815
Pridgeon, J. W., Olzmann, J. A., Chin, L.-S., and Li, L. (2007). PINK1 Protects against Oxidative Stress by Phosphorylating Mitochondrial Chaperone TRAP1. PLoS Biol. 5 (7), e172. doi:10.1371/journal.pbio.0050172
Schapira, A. H. V., Cooper, J. M., Dexter, D., Jenner, P., Clark, J. B., and Marsden, C. D. (1989). Mitochondrial Complex I Deficiency in Parkinson's Disease. Lancet 333 (8649), 1269. doi:10.1016/s0140-6736(89)92366-0
Schapira, A. H. V., Holt, I. J., Sweeney, M., Harding, A. E., Jenner, P., and Marsden, C. D. (1990). Mitochondrial DNA Analysis in Parkinson's Disease. Mov. Disord. 5 (4), 294–297. doi:10.1002/mds.870050406
Schubert, A. F., Gladkova, C., Pardon, E., Wagstaff, J. L., Freund, S. M. V., Steyaert, J., et al. (2017). Structure of PINK1 in Complex with its Substrate Ubiquitin. Nature 552 (7683), 51–56. doi:10.1038/nature24645
Sliter, D. A., Martinez, J., Hao, L., Chen, X., Sun, N., Fischer, T. D., et al. (2018). Parkin and PINK1 Mitigate STING-Induced Inflammation. Nature 561 (7722), 258–262. doi:10.1038/s41586-018-0448-9
Soutar, M. P. M., Kempthorne, L., Miyakawa, S., Annuario, E., Melandri, D., Harley, J., et al. (2018). AKT Signalling Selectively Regulates PINK1 Mitophagy in SHSY5Y Cells and Human iPSC-Derived Neurons. Sci. Rep. 8 (1), 8855. doi:10.1038/s41598-018-26949-6
Stauch, K. L., Villeneuve, L. M., Purnell, P. R., Pandey, S., Guda, C., and Fox, H. S. (2016). SWATH-MS Proteome Profiling Data Comparison of DJ-1, Parkin, and PINK1 Knockout Rat Striatal Mitochondria. Data Brief 9, 589–593. doi:10.1016/j.dib.2016.09.031
Sun, Z., Ye, J., and Yuan, J. (2022). PINK1 Mediates Neuronal Survival in Monkey. Protein Cell. 13 (1), 4–5. doi:10.1007/s13238-021-00889-w
Surmeier, D. J., Obeso, J. A., and Halliday, G. M. (2017). Selective Neuronal Vulnerability in Parkinson Disease. Nat. Rev. Neurosci. 18 (2), 101–113. doi:10.1038/nrn.2016.178
Terešak, P., Lapao, A., Subic, N., Boya, P., Elazar, Z., and Simonsen, A. (2022). Regulation of PRKN-independent Mitophagy. Autophagy 18 (1), 24–39. doi:10.1080/15548627.2021.1888244
Tolosa, E., Wenning, G., and Poewe, W. (2006). The Diagnosis of Parkinson's Disease. Lancet Neurology 5 (1), 75–86. doi:10.1016/s1474-4422(05)70285-4
Unoki, M., and Nakamura, Y. (2001). Growth-suppressive Effects of BPOZ and EGR2, Two Genes Involved in the PTEN Signaling Pathway. Oncogene 20 (33), 4457–4465. doi:10.1038/sj.onc.1204608
Valente, E. M., Abou-Sleiman, P. M., Caputo, V., Muqit, M. M. K., Harvey, K., Gispert, S., et al. (2004). Hereditary Early-Onset Parkinson's Disease Caused by Mutations in PINK1. Science 304 (5674), 1158–1160. doi:10.1126/science.1096284
Wang, K. Z. Q., Steer, E., Otero, P. A., Bateman, N. W., Cheng, M. H., Scott, A. L., et al. (2018). PINK1 Interacts with VCP/p97 and Activates PKA to Promote NSFL1C/p47 Phosphorylation and Dendritic Arborization in Neurons. eNeuro 5 (6), 0466–518. doi:10.1523/eneuro.0466-18.2018
Wang, X., Cao, C., Huang, J., Yao, J., Hai, T., Zheng, Q., et al. (2016). One-step Generation of Triple Gene-Targeted Pigs Using CRISPR/Cas9 System. Sci. Rep. 6, 20620. doi:10.1038/srep20620
Wang, X., Winter, D., Ashrafi, G., Schlehe, J., Wong, Y. L., Selkoe, D., et al. (2011). PINK1 and Parkin Target Miro for Phosphorylation and Degradation to Arrest Mitochondrial Motility. Cell. 147 (4), 893–906. doi:10.1016/j.cell.2011.10.018
Yamada, T., Dawson, T. M., Yanagawa, T., Iijima, M., and Sesaki, H. (2019). SQSTM1/p62 Promotes Mitochondrial Ubiquitination Independently of PINK1 and PRKN/parkin in Mitophagy. Autophagy 15 (11), 2012–2018. doi:10.1080/15548627.2019.1643185
Yang, W., Guo, X., Tu, Z., Chen, X., Han, R., Liu, Y., et al. (2022). PINK1 Kinase Dysfunction Triggers Neurodegeneration in the Primate Brain without Impacting Mitochondrial Homeostasis. Protein Cell. 13 (1), 26–46. doi:10.1007/s13238-021-00888-x
Yang, W., Li, S., and Li, X.-J. (2019a). A CRISPR Monkey Model Unravels a Unique Function of PINK1 in Primate Brains. Mol. Neurodegener. 14 (1), 17. doi:10.1186/s13024-019-0321-9
Yang, W., Liu, Y., Tu, Z., Xiao, C., Yan, S., Ma, X., et al. (2019b). CRISPR/Cas9-mediated PINK1 Deletion Leads to Neurodegeneration in Rhesus Monkeys. Cell. Res. 29 (4), 334–336. doi:10.1038/s41422-019-0142-y
Yang, Y., Gehrke, S., Imai, Y., Huang, Z., Ouyang, Y., Wang, J.-W., et al. (2006). Mitochondrial Pathology and Muscle and Dopaminergic Neuron Degeneration Caused by Inactivation of Drosophila Pink1 Is Rescued by Parkin. Proc. Natl. Acad. Sci. U.S.A. 103 (28), 10793–10798. doi:10.1073/pnas.0602493103
Yin, P., Li, S., Li, X. J., Yang, W., et al. (2022). New Pathogenic Insights from Large Animal Models of Neurodegenerative Diseases. Protein Cell. doi:10.1007/s13238-022-00912-8
Zhang, R., Gu, J., Chen, J., Ni, J., Hung, J., Wang, Z., et al. (2017). High Expression of PINK1 Promotes Proliferation and Chemoresistance of NSCLC. Oncol. Rep. 37 (4), 2137–2146. doi:10.3892/or.2017.5486
Zhao, Y., Qin, L., Pan, H., Liu, Z., Jiang, L., He, Y., et al. (2020). The Role of Genetics in Parkinson's Disease: a Large Cohort Study in Chinese Mainland Population. Brain 143 (7), 2220–2234. doi:10.1093/brain/awaa167
Zhou, X., Xin, J., Fan, N., Zou, Q., Huang, J., Ouyang, Z., et al. (2015). Generation of CRISPR/Cas9-mediated Gene-Targeted Pigs via Somatic Cell Nuclear Transfer. Cell. Mol. Life Sci. 72 (6), 1175–1184. doi:10.1007/s00018-014-1744-7
Keywords: PINK1, mitophagy, mitochondria, Parkinson’s disease (PD), parkin (PARK2)
Citation: Chen X, Wang Q, Li S, Li X-J and Yang W (2022) Mitochondrial-Dependent and Independent Functions of PINK1. Front. Cell Dev. Biol. 10:954536. doi: 10.3389/fcell.2022.954536
Received: 27 May 2022; Accepted: 16 June 2022;
Published: 08 July 2022.
Edited by:
Yongye Huang, Northeastern University, ChinaReviewed by:
Eszter Dombi, University of Oxford, United KingdomXiangnan Zhang, Zhejiang University, China
François Singh, University of Dundee, United Kingdom
Copyright © 2022 Chen, Wang, Li, Li and Yang. This is an open-access article distributed under the terms of the Creative Commons Attribution License (CC BY). The use, distribution or reproduction in other forums is permitted, provided the original author(s) and the copyright owner(s) are credited and that the original publication in this journal is cited, in accordance with accepted academic practice. No use, distribution or reproduction is permitted which does not comply with these terms.
*Correspondence: Weili Yang, weiliyang12@jnu.edu.cn