The cause–effect relation of tuberculosis on incidence of diabetes mellitus
- 1Laboratory of Molecular Cell Biology, Centre for DNA Fingerprinting and Diagnostics (CDFD), Hyderabad, India
- 2Regional Centre for Biotechnology, Faridabad, India
- 3Molecular Biology Unit, Indian Council of Medical Research (ICMR)-National Institute of Nutrition, Jamai Osmania PO, Hyderabad, India
Tuberculosis (TB) is one of the oldest human diseases and is one of the major causes of mortality and morbidity across the Globe. Mycobacterium tuberculosis (Mtb), the causal agent of TB is one of the most successful pathogens known to mankind. Malnutrition, smoking, co-infection with other pathogens like human immunodeficiency virus (HIV), or conditions like diabetes further aggravate the tuberculosis pathogenesis. The association between type 2 diabetes mellitus (DM) and tuberculosis is well known and the immune-metabolic changes during diabetes are known to cause increased susceptibility to tuberculosis. Many epidemiological studies suggest the occurrence of hyperglycemia during active TB leading to impaired glucose tolerance and insulin resistance. However, the mechanisms underlying these effects is not well understood. In this review, we have described possible causal factors like inflammation, host metabolic changes triggered by tuberculosis that could contribute to the development of insulin resistance and type 2 diabetes. We have also discussed therapeutic management of type 2 diabetes during TB, which may help in designing future strategies to cope with TB-DM cases.
1 Introduction
Tuberculosis (TB) is a major health burden in the world. About 10.6 million people fell ill with TB and approximately 1.6 million deaths have occurred in the year 2021 (World Health Organization, 2022). The severity of the disease is further increased by malnutrition, poverty, smoking, alcohol abuse, and co-infection with other diseases like HIV (human immunodeficiency virus) and diabetes (Yorke et al., 2017). The association of tuberculosis with HIV and diabetes mellitus (DM), further complicates TB treatment (Baker et al., 2011; Piggott and Karakousis, 2011; Niazi and Kalra, 2012). Diabetes is a complex metabolic disorder characterized by elevated blood glucose levels due to insulin resistance, insufficient insulin production or both (Sami et al., 2017). Majority of the diabetic patients can be classified into two broad categories, type 1 and type 2. The type 1 diabetes is mostly related to autoimmune destruction of pancreatic β-cells resulting in absolute or near absolute failure of insulin production and secretion. It constitutes about 5% - 10% of total diabetic population. Whereas type 2 diabetes is related to insulin resistance (Gharravi et al., 2018; Tan et al., 2019). Uncontrolled diabetes can result in serious consequences like diabetic nephropathy (kidney dysfunction), pancreatic inflammation (leading to persistent hyperglycaemia), liver damage (cirrhosis and liver failure), inflammatory gut, and insulin resistance in liver and skeletal tissue (Daryabor et al., 2020; Selby and Taal, 2020). Tuberculosis and diabetes mellitus co-occurrence has been observed in about 0.4 million people in 2021 (World Health Organization, 2022). It is debatable whether active tuberculosis increases the risk of diabetes, however, various studies suggest that active TB can cause impaired glucose tolerance (IGT) which may lead to onset of type 2 diabetes (Mugusi et al., 1990; Oluboyo and Erasmus, 1990; Jeon et al., 2010). It is reported that impaired blood glucose tolerance can be normalized after the successful treatment of TB, but it probably persists as a risk factor for developing type 2 diabetes mellitus in the future (Yorke et al., 2017; Krishnappa et al., 2019). Several studies suggest that 5% - 30% of TB patients have concomitant diabetes mellitus and the rise in diabetic patients is highly predominant in poor and developing countries where the TB epidemic is more widespread, making TB-DM co-occurrence more frequent (Nichols, 1957; Mugusi et al., 1990; Nijland et al., 2006; Ruslami et al., 2010; Niazi and Kalra, 2012; Deng et al., 2016; Atlas IDFD, 2021; World Health Organization, 2022). India, Indonesia, China, Philippines Pakistan, Nigeria, Bangladesh, and Democratic Republic of Congo contribute to more than 2/3rd of world TB population in 2021 and are among the highest growth rate of diabetic patients (Atlas IDFD, 2021; World Health Organization, 2022). The International Diabetes Federation (IDF) has reported that the current worldwide diabetes disease burden is about 537 million, which can hit 643 million by 2030 and 783 million by 2045 (Atlas IDFD, 2021). Evidence indicating active TB disease may influence the pathogenesis of insulin resistance and incidence of type 2 diabetes (Guptan and Shah, 2000; Jeon et al., 2010; Workneh et al., 2017; Magee et al., 2018; Menon et al., 2020). Interestingly, latent TB can also influence type 2 diabetes incidents and about 25 global population have latent TB condition (Magee et al., 2022) as well as type 2 diabetes can also be a risk factor for the acquisition of latent TB infection (Lee et al., 2017; Ssekamatte et al., 2021). The combination of TB and DM (TB-DM) may interfere with the therapeutic intervention of TB as well as DM and can influence the disease course. There is a high burden of DM among TB patients at global level, however, the global prevalence of TB among DM patients is low (Workneh et al., 2017). Therefore, it is important to understand the factors that contribute to effective TB-DM therapeutic management. This review will emit light on correlation of tuberculosis and diabetes, and the possible causal factors responsible for occurrence of DM in TB patients.
2 TB-DM complications and DM susceptibility in TB patients
TB-DM co-occurrence may cause adverse effect on TB treatment and may subsequently increase the multi-drug resistance incidence (Baker et al., 2011; Tegegne et al., 2018). Delayed sputum conversion from smear-positive to smear-negative and higher treatment failure was observed in TB-DM patients as compared to non-diabetic TB patients (Viswanathan et al., 2014). Further studies showed that the mortality rate was higher in tuberculosis patients with DM and the MDR-TB rate was 1.6 to 3.8 folds higher in TB-DM patients as compared to TB alone (Workneh et al., 2016; Evangelista et al., 2020). However, many reports have shown that TB makes ground for diabetes or pre-diabetes conditions (Yorke et al., 2017). In most cases, these pre-diabetic symptoms turn to normal after successful tuberculosis treatment (Oluboyo and Erasmus, 1990) but frequently hyperglycemia and type 2 diabetes is observed as a side-effect of TB (Magee et al., 2018). The prevalence of DM among tuberculosis patients in Ethiopia was 74 out of 1000 patients (Omar et al., 2021) and in India (second highest diabetic patients in the world [Atlas IDFD, 2021]), the prevalence of diabetes among TB patients was 25.3% and that of pre-diabetes was 24.5% (Viswanathan et al., 2012). The prevalence of diabetes mellitus among TB patients in South India was shown to be 39%, indicating one in three TB patients had co-existing DM (Rajaa et al., 2021). TB epigenetically enhances DM-linked complication pathways (Prada-Medina et al., 2017). Thus, it is very important to understand the factors/mechanisms that are responsible for occurrence of DM in TB patients (Atlas IDFD, 2021; World Health Organization, 2022). Various factors and cellular signaling cascades that can lead to the development of diabetic/pre-diabetic conditions in TB patients are described below.
2.1 Insulin resistance
Insulin regulates blood glucose levels through glucose and lipid metabolism in the body. Insulin resistance is clinically defined as a condition where known amount of exogenous or endogenous insulin fails to increase glucose uptake and utilization as much as in a normal population (Lebovitz, 2001). In other words, the body develops diminished response to the actions of insulin or impaired sensitivity to insulin resulting in higher blood glucose levels. Insulin resistance is one of the pre-diabetic conditions that can lead to diabetes and metabolic syndromes (Suzuki et al., 1996; Ormazabal et al., 2018). IR is often associated with tuberculosis and is suggested to be a risk factor as well as a potential marker for active tuberculosis (Mao et al., 2011, Yurt et al., 2013). A cross-section cohort study in South Africa showed correlation between TB and IR in newly diagnosed TB patients which gets reverted after successful treatment (Philips et al., 2017). The overall (both male and female) prevalence of IR in this study was 25.4% (Philips et al., 2017). It has been shown that Mtb-infected mice are more susceptible to develop hyperglycaemia and insulin resistance than uninfected mice and this susceptibility is further increased with high-fat diet and age (Oswal et al., 2022). Mtb infection causes dysregulation of lipid metabolism and elevates the levels of circulating free fatty acids which causes ectopic deposition of lipids in organs important for glucose homeostasis like liver and skeletal muscles leading to development of IR (Badin et al., 2011; Oswal et al., 2022). Multiple studies suggest that TB-DM/IR incidents occur with altered lipid metabolism that includes high LDL (low-density lipoprotein) cholesterol, low HDL (high-density lipoprotein) cholesterol, high levels of very low-density lipoprotein (VLDL) triglycerides, resulting in severe clinical manifestation and disease outcome (Vrieling et al., 2018; Shvets et al., 2021). IR was also observed during the early phase of anti-tuberculosis therapy which can be attributed to impaired liver function due to the toxic effect of first-line anti-tuberculosis drugs like isoniazid, rifampicin and pyrazinamide (Shvets et al., 2019). Insulin resistance results in abnormalities in PI3K/Akt pathway causing wasting in muscle tissue which is also observed in tuberculosis patients (Wang et al., 2006; Mupere et al., 2014).
2.2 Hyperglycaemia
Tuberculosis patients generally have temporal hyperglycaemic conditions. Among the patients that are hyperglycaemic during the start of TB treatment, a high proportion of patients receive a new diabetes diagnosis without prior DM history (Magee et al., 2018). The incidence of hyperglycaemia in tuberculosis may be attributed to stress, prolonged inflammation, change in the glucose and lipid metabolism of TB patients and insulin resistance syndrome (Guptan and Shah, 2000; Magee et al., 2018; Menon et al., 2020). A clinical study in India suggests that 7% tuberculosis patients had DM and 4.5% patients have IGT or impaired blood glucose tolerance and about 65% of IGT patients could revert to normoglycemic conditions after completion of anti-TB therapy (Krishnappa et al., 2019). There is a study in Periurban South Africa that shows an association between transient hyperglycemia, and TB-DM/TB-IGR (impaired glucose regulation) in newly diagnosed DM patients as well as an association between persistent hyperglycemia and TB-DM in patients with HIV-1, despite TB therapy (Kubjane et al., 2020). A meta-analysis investigation showed that 27.3% TB patients had newly developed hyperglycaemic condition at baseline level and after a follow-up of 3 - 6 months, 50% of the hyperglycaemic conditions remained unresolved (Menon et al., 2020). In a follow-up study in Brazil with HIV-positive TB patients, Moreira et al. (2018) had indicated that hyperglycemia frequently occurs in HIV-infected patients who initiate TB treatment, and it increases the risks of adverse TB outcomes (Moreira et al., 2018). Another screening study for diabetes among TB patients in India showed the prevalence of newly diagnosed diabetes was approximately 10.8% where HbA1c ≥ 47.5 mmol/mol was used for diagnosis of diabetes (Kumpatla et al., 2013). Various studies reveal that the prevalence of hyperglycaemia in tuberculosis patients varies between 10% to 26% which depends on age, sex and fasting blood glucose level (Moreira et al., 2018; Menon et al., 2020; Ephraim et al., 2021). An interesting study indicated that hyperglycaemia is associated with increased risk of patient delay in pulmonary tuberculosis which is a serious concern in the context of diagnosis of TB, increased risk of transmission of TB infection in the community as well as clinical manifestation of TB (Wang et al., 2017). Liver toxicity during tuberculosis is often associated with the first line anti-TB drugs which increase hepatic enzyme levels and may be responsible for hyperglycemia in diabetic patients (Molla et al., 2021). TB-DM and TB patients have high levels of liver enzymes compared to normal (Sama et al., 2017). The hyperglycemic response against anti-TB drugs like rifampicin may enhance the requirement for insulin in type 1 diabetes patients (Atkin et al., 1993). Thus, rifampicin disturbs glycemic control in both type 1 and type 2 diabetes.
2.3 Inflammation
Inflammation is critical for controlling infection and a dysregulation inflammatory response is central to pathogenesis of several pathogens including tuberculosis. The ability of M. tuberculosis to modulate the fundamental inflammatory processes such as recruitment of immune cells to the site of infection, prodcution of pro-inflammatory cytokines, production of eicosanoids and lipid mediators associated with inflammation favors its survival inside the host (Kaufmann and Dorhoi, 2013). Inflammation during active tuberculosis results in an environment with higher pro-inflammatory cytokine production as a protective response in host against the bacilli. Inflammation is nonresolving in nature in both active and latent tuberculosis (Kaufmann and Dorhoi, 2013), characterized by elevated levels of pro-inflammatory cytokines (He et al., 2020) which often lead to metabolic dysregulation and eventually type 2 diabetes (Spranger et al., 2003; Das and Mukhopadhyay, 2011; Tsalamandris et al., 2019). On the other hand, the hyperglycaemic environment often results in dysfunction of the immune system like inhibition of leukocyte recruitment, damage to the neutrophil function, macrophage dysfunction, depression of the antioxidant system and humoral immunity as well as modulation of inflammatory signaling cascades causing the DM patients to be susceptible to various infections (Casqueiro et al., 2012; Berbudi et al., 2020).
Co-incidence of pulmonary TB with pre-diabetes causes elevated type-I, type-II, and Th17 cytokine responses with higher circulating levels of interferon-gamma (IFN-γ), IFN-β, tumor necrosis factor-alpha (TNF-α), interleukin (IL)-2, IL-5, IL-17A, IL-17F, IL-10, IL-1β, IFN-β, transforming growth factor-β (TGF-β) and Granulocyte-macrophage colony-stimulating factor (GM-CSF), however, no significant correlation was observed between Mtb bacterial burden and cytokine levels (Kumar et al., 2014).
Toll-like receptors (TLRs) are members of the innate immunity that works as pathogen-associated molecular pattern (PAMP) receptors and play a crucial role in eliciting innate immune response which dictate subsequent adaptive immune responses (Akira and Takeda, 2004). Activation of TLRs usually triggers a signaling cascade which results in the production of pro-inflammatory cytokines/chemokines through nuclear factor kappa B (NF-κB) (Udgata et al., 2019). Several mycobacterial components are recognized by TLR2 and TLR4 (Means et al., 1999; Heldwein and Fenton, 2002). Several lines of evidences point to a role of TLRs in the development of insulin resistance and diabetes. Among the TLRs, TLR2 and TLR4 expression have been shown to be increased in conventional insulin resistance target tissues like skeletal muscle and adipose tissue of type 2 diabetic subjects (Creely et al., 2007; Reyna et al., 2008). Song et al. (2006) showed that TLR4 triggering leads to NF-κB activation and expression of TNF-α, IL-6, and resistin which have been implicated for the development of insulin resistance in adipocytes. Type 2 diabetic subjects were found to have significantly increased TLR2, TLR4 mRNA, and protein in monocytes and increased TLR2 and TLR4 expression was correlated with BMI, homeostasis model assessment–insulin resistance (HOMA-IR), glucose, A1C, Nϵ-(carboxymethyl) lysine (CML), and free fatty acid (FFA) (Dasu et al., 2010). Nonetheless, the expression levels of TLR2 were found to be upregulated in obese individuals who are at higher risk of development of insulin resistance as obese type 2 diabetes patients had higher expressions of TLR2 in comparison to obese patients without type 2 diabetes (Ahmad et al., 2012).
Interestingly, signaling through TLR2 can elicit both pro- and anti-inflammatory response depending on the nature and site of interaction with its ligand on the leucine rich repeat (LRR) motif present on the ectodomain of TLR molecule (Udgata et al., 2019). It has been found that a member of the PPE family of Mycobacterium tuberculosis, PPE18 interact with LRR 11~15 on TLR2 ectodomain and induces IL-10 production from macrophages and elicits anti-inflammatory responses (Nair et al., 2009). In contrast, PPE17 protein of Mtb was found to specifically interact with TLR2 LRR 15~20 domain to activate NF-κB and induced pro-inflammatory-type signaling (Bhat et al., 2012). Also, it has been shown that the cellular localization of heat shock protein 60 of Mtb (Mtbhsp60) upon interaction with TLR4 can dictate the inflammatory responses in macrophages (Parveen et al., 2013). Thus, there is a possibility of induction of insulin resistance by Mtb using PPE17/Mtbhsp60 by targeting the TLR-NF-κB-triggered signaling cascades. Interestingly, it has been shown that Mycobacterium sp. utilizes TLR2 to promote inflammation (Kanagawa et al., 2015). Mycobacterial secretory protein MTP53 (Rv2878c), a disulphide bond-like forming protein also induces TGF-β-activated kinase 1-mediated pro-inflammatory cytokine response during Mtb infection (Wang et al., 2019; Pal et al., 2022). Some lipases of Mtb can also trigger inflammatory responses and modulate immune responses (Rameshwaram et al., 2018). For example, Rv0183 is involved in triggering MyD88-NF-κB signaling and induction of inflammatory molecules like IL-6, TLR2, TLR6 and TNF-α (Xu et al., 2010). Another lipase LipC can hydrolyze short-chain esters and elicits cytokines/chemokines induction (Shen et al., 2012). The lipases have an adverse effect on patients, as they cause the production of inflammatory cytokines as well as generates excess free fatty acids/lipid mediators. Excess free fatty acids get deposited in tissues important for glucose homeostasis like liver and muscle to cause systemic insulin resistance that may lead to insulin resistance (Martinez et al., 2019).
Inflammation also plays a key role in the development of insulin resistance and T2D during obesity as deletion of jun kinase 1 (JNK1, a key negative regulator of insulin sensitivity in the obese state) in the nonhematopoietic compartment protects mice against HFD-induced insulin resistance and T2D by inhibiting HFD-induced inflammation (Solinas et al, 2007; Das and Mukhopadhyay, 2011). Inflammation changes the expression of key genes of adipose tissue including SREBP-1, Resistin, RANTES, leptin, and adiponectin involved in glucose homeostasis. The sterol regulatory element binding protein-1 (SREBP-1) regulates genes that are involved in fatty acid synthesis and lipogenesis during inflammation. TNF-α completely blocks SREBP-1 expression and its activation (Sewter et al., 2002; Das and Mukhopadhyay, 2011). Resistin also links to inflammation and higher resistin levels were observed in tuberculosis and T2D patients (Youn et al., 2004; Gharibeh et al., 2010; Das and Mukhopadhyay, 2011; Moideen et al., 2018). Resistin activates pro-inflammatory cytokines like IL-12 and TNF-α through the NF-κB-dependent pathway in mouse and human macrophages and is directly correlated to glucose homeostasis (Asensio et al., 2004; Silswal et al., 2005). RANTES also known as CCL5 is an adipokine secreted by adipocytes and involved in the recruitment of macrophages, T cells, eosinophils, and basophils (Appay and Rowland-Jones, 2001; Keophiphath et al., 2010) and cause inflammation. RANTES also impaired glucose-induced insulin secretion in mice by reducing the glucose-dependent secretion of glucagon-like peptides (Pais et al., 2014). RANTES is also involved in insulin responsiveness through CCR5, which regulates insulin signaling in the hypothalamus and contributes to systemic insulin sensitivity and glucose metabolism (Chou et al., 2016).
2.4 Adipose tissue
Adipose tissue functions as an endocrine organ by regulating metabolism and inflammation through secretion of adipokines, cytokines, peptides, and release of free fatty acids. Reports indicate that adipose tissue inflammation is consistently associated with excess fat mass and insulin resistance and development of type 2 diabetes (Guilherme et al., 2008; Burhans et al., 2019). Mtb infection in adipose tissue causes adipocyte hypertrophy, lipolysis, alterations in metabolic processes, and infiltration of immune cells (Ayyappan et al., 2019). Hypertrophic or enlarged adipocytes are characterized by altered secretion patterns of adipokines, as well as secretion of higher levels of pro-inflammatory cytokine like TNF-α and MCP-1, which not only impair insulin signaling in the skeletal muscle and liver but also induce systemic insulin resistance (Guilherme et al., 2008). Adipose tissue produces chemokine monocyte chemoattractant protein-1 (MCP-1/CCL2) and leptin which are strongly associated with inflammation. The adipokine MCP-1 promotes inflammation and diabetic nephropathy (Tesch, 2008). MCP-1 production is higher in adipose tissue and CD14+ monocytes in TB patients. MCP-1 is a potent pro-inflammatory molecule that plays a critical role in inducing inflammatory response during TB by attracting monocytes and T lymphocyte (Lin et al., 1998; Martinez et al., 2019). MCP-1 overexpression causes insulin resistance in adipose tissue and steatosis in the liver during obesity. Mtb-infected visceral adipose tissue has increased MCP-1 expression which alters adipocyte function (Erol, 2008). It is suggested that MCP-1 probably induces adipocyte dedifferentiation and adds to pathologies associated with hyperinsulinemia, insulin resistance and obesity, including type 2 diabetes (Sartipy and Loskutoff, 2003; Erol, 2008; Panee, 2012). Interestingly, it has been noted that addition of MCP-1 to differentiated adipocytes in vitro results in downregulation of insulin-stimulated glucose uptake as well as expression of various genes like LpL, adipsin, GLUT-4, aP2, beta3-adrenergic receptor and peroxisome proliferator-activated receptor gamma (PPAR-γ) (Sartipy and Loskutoff, 2003).
Mtb-infected adipose tissue recruits myeloid and NK-cell lineage which produce higher levels of TNF-α, IL-12 and other inflammatory molecules (Erol, 2008; Beigier-Bompadre et al., 2017). TNF-α binds to TNFR on adipocytes and increase production of intracellular cAMP that activates protein kinase A-induced lipolysis and generates free fatty acids (Zhang et al., 2002; Song et al., 2006). The lipolytic activity of TNF-α is shown to be influenced by glucose in adipocytes (Green et al., 2004). The increased free fatty acids released by the adipocytes are ectopically deposited in various organs like liver, skeletal muscles, pancreas and gastrointestinal tract causing lipotoxicity (Sears and Perry, 2015). In liver, free fatty acid increases TAG formation/accumulation (liver steatosis), increase hepatic glucose production (increased gluconeogenesis and glucogenolysis) and insulin resistance leading to hyperglycemia (Boden, 2003; Sears and Perry, 2015). Free fatty acid accumulation in skeletal muscles inhibit glucose uptake, reduce fatty acid oxidation and reduce insulin sensitivity which, results in insulin resistance (Delarue and Magnan, 2007; Lyons et al., 2016). The physiological changes and modulation in the signaling cascades in the adipose tissue during Mtb infection that can lead to insulin resistance is described in Figure 1.
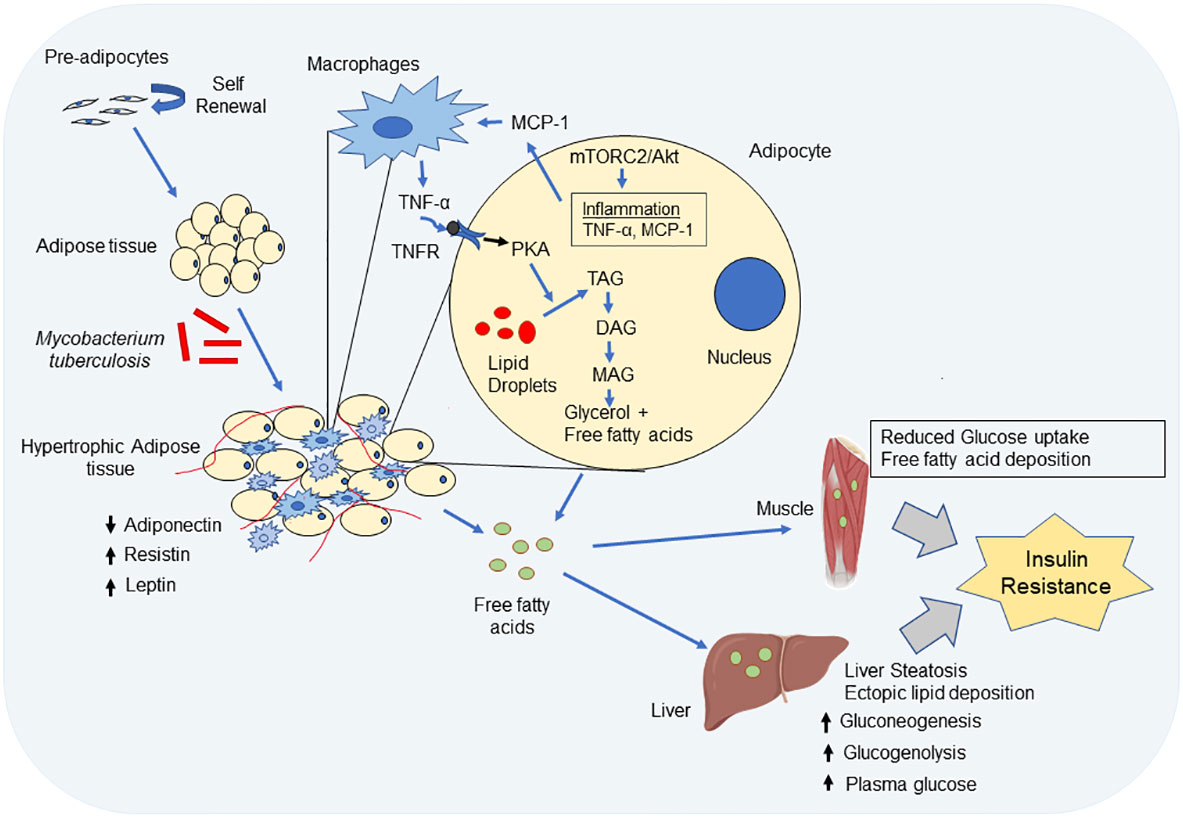
Figure 1 Impact of Mycobacterium tuberculosis (Mtb) infection on the physiological functions of adipocyte. Mtb infection leads to hypertrophy and immune cell infiltration into adipose tissue. Hypertrophic adipose tissue decreases expression of adiponectin, but increases expression of leptin and resistin. Decreased adiponectin expression reduces insulin sensitivity and through simultaneous upregulation of leptin and resistin, there is induction of inflammation and insulin resistance. Again, through mTORC2/Akt activation in adipocytes, Mtb bacteria cause inflammation and induce TNF-α and monocyte chemoattractant factor-1 (MCP-1) and reduce adiponectin. MCP-1 recruits macrophages and other cell type into adipose tissue. The infiltrated immune cells in adipocyte further produce inflammatory cytokine like TNF-α and IL-6. TNF-α binds to TNF receptor on adipocyte and induce activation of protein kinase A which phosphorylates hormone sensitive lipase and causes lipolysis in adipocytes. The inflammation in adipose tissue increased lipolysis, i.e. degradation of tri-acylglycerol (TAG) to di-acyleglycerol (DAG), mono-acylglycerol (MAG) and finally glycerol and release of free fatty acids (FFA). FFA through circulation gets deposited in other sites like liver and muscle. In liver, fat deposition leads to increase gluconeogenesis, gluconeolysis and leads to liver steatosis which results in increased plasma glucose concentration and finally leads to insulin resistance. Free fatty acid deposition in muscle tissue also causes insulin resistance in muscles.
Mycobacterium tuberculosis can activate macrophages through various mechanisms like binding of pathogen associated molecular patterns (PAMPs) to the Toll like receptors (TLRs) present on the macrophages to induce production of pro-inflammatory cytokines (Dolasia et al., 2018), production of reactive oxygen species (ROS) by NADPH oxidase complex and reactive nitrogen intermediates (RNI) (Nathan and Shiloh, 2000), modulation of signaling pathways such as MAPK, NF-κB pathway (Cambier et al., 2014; Mayer-Barber and Sher, 2015). These activated macrophages can secrete various pro-inflammatory cytokines like TNF-α, IL-6 (Lumeng and Saltiel, 2011) which can lead to extensive remodeling of the adipose tissue (Sun et al., 2011; Hotamisiligil, 2017). One mechanism by which macrophage activation may contribute to adipocyte hypertrophy is through the secretion of matrix metalloproteinases (MMPs) which degrade extracellular matrix components, allowing adipocytes to expand in size (Lijnen et al., 2002). Macrophages can secrete MMPs, particularly MM2 and MMP-9, in response to M. tuberculosis infection (Quiding-Järbrink et al., 2001). On the other hand, levels of adipokines like resistin were found to be increased in pulmonary TB patients (Chang et al., 2013). Resistin is known to increase lipolysis in the visceral adipose tissue (Chen et al., 2014) by increasing expression of hormone sensitive lipase (HSL) leading to increased levels of serum free fatty acids (Qatanani et al., 2009) which cause a positive feedback loop that may contributes to the development of adipocyte hypertrophy (Pérez-Torres et al., 2019).Leptin is another important adipose-derived hormone, which regulates food intake and body weight, and is expressed by adipocytes of white adipose tissue (Kelesidis et al., 2010). Lower body fat and wasting in TB patients is probably associated with lower serum leptin concentrations and a gradual increase in leptin levels is observed post-TB treatment (vanCrevel et al., 2002). Also prolonged inflammation during tuberculosis may be responsible for decreased leptin production (vanCrevel et al., 2002). Leptin deficiency is shown to cause severe insulin resistance and related endocrine disorders in uncontrolled insulin-deficient diabetes (German et al., 2010).
2.5 Lipids and free fatty acids
Lipids and their mediators are important components of innate immunity against M. tuberculosis (Chen et al., 2008). The modulation in lipid profile can cause atherosclerosis, insulin resistance (Laakso et al., 1990) and other cardiovascular diseases (Dong et al., 2021). Mtb persists inside adipose tissue and alters its metabolic properties to modulate fatty acid metabolism (Neyrolles et al., 2006; Erol, 2008). Lipolysis in adipose tissue is another effect of tuberculosis that causes generation of low HDL and higher levels of total cholesterol, LDL, very low-density lipoprotein and non-esterified serum free fatty acids (NEFA) (Vrieling et al., 2018; Ayyappan et al., 2019). High serum triglyceride levels and free fatty acids result in the deposition of lipid granules in various organs, that is known to inhibit insulin-associated signaling cascades resulting in insulin resistance (Boden and Shulman, 2002; Xin et al., 2019). Lipid profile abnormalities and an increased risk of diabetes were observed in multidrug-resistant (MDR) TB patients (Biranu et al., 2021). Lipid mediator, lipoxin and leukotriene derived from arachidonic acid is associated with Mtb susceptibility and disease pathogenicity (Mayer-Barber and Sher, 2015). Interestingly, it has been observed that leukotriene B4 plays a central role in systemic inflammation and establishment of insulin resistance in animal model of diabetes (Li et al., 2015). Secretory mycobacterial lipase Rv0183 is shown to be involved in degradation of lipid bodies present in adipocyte or foamy macrophages causing generation of free fatty acids which may be responsible for metabolic syndrome during tuberculosis (Erol, 2008; Dhouib et al., 2010; Dedieu et al., 2013).
Persistent chronic inflammation is considered one of the major risk factors for development of cardiovascular diseases (Ferrucci and Fabbri, 2018) and systemic inflammation levels have been associated with sputum bacterial load (Mesquita et al., 2016). Interestingly, tuberculosis and cardiovascular diseases were found to have a close epidemiological connection (Huaman et al., 2015). It has been found that granuloma formation during tuberculosis can cause coronary arteritis leading to myocardial infarction (Rodríguez et al., 2012). In addition, production of pro-inflammatory cytokines by activated macrophages and CD4+ T cells can also contribute to development of cardiovascular complications (Huaman et al., 2015). The hallmarks of atherosclerosis are LDL oxidation, foam cell formation and inflammation (Chistiakov et al., 2017). Tuberculosis patients have been shown to have higher susceptibility to LDL oxidation and lipid peroxidation (Nezami et al., 2011) leading to formation of foam cell macrophages (Agarwal et al., 2021) which have been implicated to play a role in the development of TB granulomas and persisting Mtb infection. Diabetes is also often associated with dyslipidemia characterized by increased VLDL, LDL-cholesterol (LDL-C) and decreased high-density lipoprotein cholesterol (HDL-C) (Schofield et al., 2016) and the levels of oxidized LDL (oxLDL) were found to be significantly higher in diabetic patients (Banerjee et al., 2019). Uptake of oxLDL mediated by scavenger receptor CD36 accounts for a large proportion of the formation of macrophage foam cells and formation of atherosclerotic plaques (Luo et al., 2017). Furthermore, patients with concurrent TB and diabetes have been shown to have pro-atherogenic plasma lipid profile with significantly elevated levels of sphingomyelins and remnant-like lipoprotein particles (Vrieling et al., 2018). Therefore, M. tuberculosis infection and diabetes can independently contribute to the development of atherosclerosis.
2.6 Host metabolic changes
Mtb proteins can affect host glucose and lipid metabolism to create a favourable condition for the survival of the bacilli. It is reported that pre-diabetic condition is favourable for survival of Mtb bacilli (Sinha et al., 2021). Some Mtb proteins are shown to modulate host metabolism to induce a favourable niche. For example, ESAT-6 protein of Mtb induces GLUT-1-mediated glucose uptake in macrophages which perturbs glycolytic pathway and causes foamy macrophages formation (Singh et al., 2015). Interestingly, Mtb-infected macrophages have increased glucose uptake, upregulated expression of glycolytic enzymes and downregulated levels of enzymes of tricarboxylic cycle and oxidative phosphorylation which results in lactate production causing the Warburg effect. (Shi et al., 2015; Howard and Khader, 2020). This results in decreased rate of mitochondrial respiration which causes inflammation in macrophages and adipocytes which is likely to further contribute to subsequent systemic insulin resistance (Wang et al., 2020). Mtb can utilize lactate with L-lactate dehydrogenase gene, lldD2 (Rv1872c) and facilitate its intracellular survival. It has been shown that lldD2 knock-out Mtb fails to efficiently replicate in human macrophages (Billig et al., 2017). The tuberculosis necrotizing toxin (TNT) of Mtb hydrolyses NAD+ and causes generation of reactive oxygen species (ROS) which is a risk factor for type 2 DM (Pajuelo et al., 2020). In M1-polarized macrophages, the TLR/PRR-triggered signaling causes NF-κB-induced inflammation and production of hypoxia mediated factor-1α (HIF-1α) (Corcoran and O'Neill, 2016; Dorrington and Fraser, 2019). HIF-1α induces lipid body formation, upregulation of glycolytic genes and inflammatory mediator like IL-1β (Howard and Khader, 2020). Increased glycolysis causes pyruvate production which is transported into the mitochondria and forms 3 hydroxybutyrate and enters into tricarboxylic acid (TCA) cycle and causes accumulation of citrate which is transported to cytosol. Citrate stabilizes HIF-1α and is converted to Acetyl-CoA which fed into fatty acid biosynthesis. The acyl-CoA synthase long-chain family members, mainly ACSL1 and ACSL4 introduce long chain fatty acid especially arachidonic acid into membrane phospholipid (Shi et al., 2019). Cytosolic phospholipase A2 (cPLA2) release arachidonic acid from membrane phospholipids. Arachidonic acid gives rise to prostaglandin and leukotrienes which are responsible factors of inflammation. Mtb also secretes lipases that are involved in formation of lipid droplets which are risk factors for DM (Rameshwaram et al., 2018). For example, Rv2672c exhibits both lipase and proteinase activity (Singh et al., 2017). The other secretory mycobacterial Rv1083 protein is a monoacylglycerol lipase which is involved in metabolizing the host cell membrane lipids (Saravanan et al., 2012). Mtb Rv0183 produces glycerol and free fatty acids which further causes metabolic perturbations (Grininger et al., 2021). Rv1076 is another Mtb secretory esterase that hydrolyzes short carbon chain substrate (Kaur et al., 2017; Li et al., 2017). Other secretary enzymes like Rv1984c hydrolyzes medium chain carboxylic esters and monoacylglycerol whereas Rv3452 is a phospholipase A2 (Schué et al., 2010). Intracellular Mtb in macrophages can utilize host amino acids directly from cytosol for biosynthesis of its proteins (Borah et al., 2019). The metabolic reprogramming and wasting syndrome during tuberculosis follow conserved mechanism in human patients, mice and zebrafish. The concentration of circulating small amino acids is lower in human patients as well as mycobacterium-infected mice and zebrafish (Ding et al., 2020). Circulating amino acids like histidine, tryptophan, methionine, and glutamine are significantly decreased in TB patients but in case of TB-DM, other amino acids like choline, glycine, serine, threonine levels are also lower as compared to patients with TB alone (Weiner et al., 2012; Weiner et al., 2018; Vrieling et al., 2019). Histidine, glutamine (and the (E,E)-isomer of bilirubin) are shown to be negatively associated with development of type 2 diabetes and histidine-mediated suppression of hepatic glucose production is a potential target for the treatment of type 2 diabetes (Kimura et al., 2013; Peddinti et al., 2017). TNF-α secreted during Mtb infection also plays a major role to promote lipid droplet formation (Guerrini et al., 2018; Jung et al., 2020). The alteration of host metabolic processes during infection with Mtb is described in Figure 2.
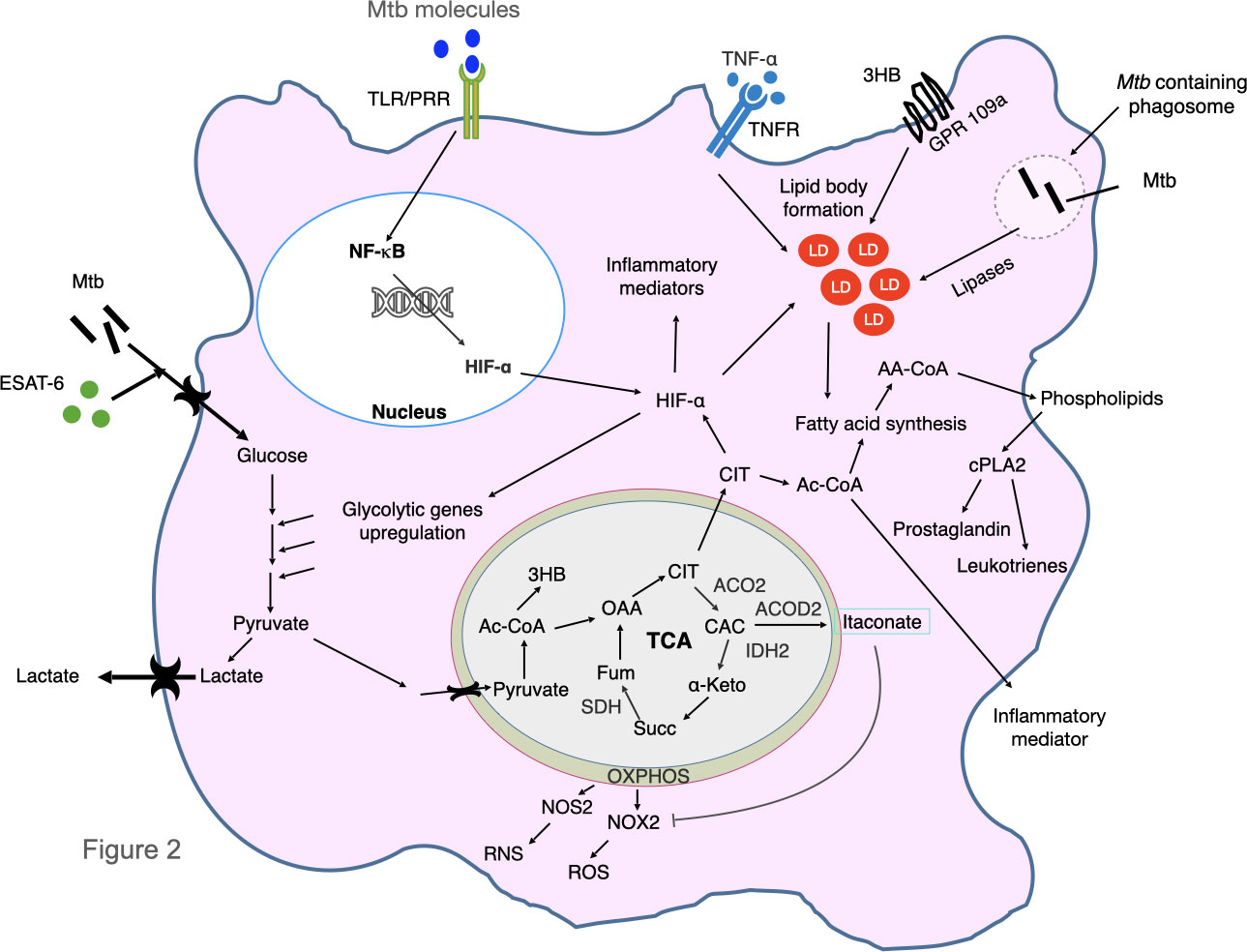
Figure 2 Mycobacterium tuberculosis infection alters host metabolic pathways. Mtb and ESAT-6 protein increase glucose uptake and up-regulate glycolytic genes and inhibit oxidative phosphorylation causing Warburg effect. This results in production of pyruvate which is converted to lactate and is secreted out, excess pyruvate is transported to mitochondria and form acetyl-CoA (Ac-CoA) and finally produce ketone bodies 3 hydroxybutyrate (3HB). 3HB binds to GPR109a and cause lipid body formation. Ac-CoA is converted to OAA and enters in the TCA (Tricarboxylic acid) cycle. The OXPHOS and TCA cycle enzymes are downregulated (that include ACO2, IDH2, ACOD2 and SDH) by Mtb. As a result, CIT is accumulated which stabilizes and increases expression of hypoxia inducible factor-α (HIF-α) and increases production of itaconate. HIF-α induce lipid body formation, upregulation of glycolysis genes and inflammatory mediator like IL-1β. Itaconate inhibits production of reactive oxygen species (ROS). Decreased OXPHOS also causes production of ROS and reactive nitrogen species (RNS). Excess CIT is converted into Ac-CoA which is utilized in the fatty acid synthesis pathway and causes formation of membrane phospholipids. Cytosolic phospholipase A2 (cPLA2) release Arachidonic acid into cytosol which gives rise to prostaglandin and leucotrienes which cause inflammation. Various toll like receptor (TLR) and pattern recognition receptor (PRR)-triggered signaling stimulation cause NF-κB activation and transcription of inflammatory mediators, as well as production of HIF-1α. M. tuberculosis in phagosome secrete various lipases which degrade host lipids that are responsible for generation of fatty acids. The TNF-α-mediated signaling is also involved in formation of lipid droplets. CAC, cis-aconitate; CIT, citrate; FUM, fumarate; α-KG, α-ketoglutarate; NOS2, nitric oxide synthase 2; NOX2, NADPH oxidase; OAA, oxaloacetate; PGE2, prostaglandin E2; SUCC, succinate; TNF, tumor necrosis factor; TNFR, tumor necrosis factor receptor; ACOD2, aconite dehydrogenase, ACO2, aconitase2, SDH succinate dehydrogenase, IDH2, isocitrate dehydrogenase 2, LD, lipid droplets, OXPHOS, oxidative phosphorylation, AA-CoA, acyl Co-A.
In conclusion, active TB itself can induce various immune-metabolic changes like increased inflammation, adipose tissue modulation, increased free fatty acid levels leading to development of insulin resistance in patients that can lead to development of type 2 diabetes if not managed clinically. Irregular lipolysis in adipose tissue, altered glucose and lipid metabolism in adipose tissue and other organs including lungs due to increased accumulation of intracellular lipids as well as inflammatory milieu around the adipose tissue are possible reasons for development of IR during tuberculosis (Oswal et al., 2022). However, further studies are needed to find out the exact molecular mechanism of development of IR in TB patients. Prolonged hyperglycaemia can be considered as a risk factor for DM and cardiovascular diseases. The host immune response to active TB disease results in a prolonged state of systemic inflammation characterized by activation of various immune cells by binding with PAMPs through the TLRs resulting in elevated levels of pro-inflammatory cytokines like IL-1β and IL-6 which are known to directly inhibit insulin-stimulated glucose uptake and thus implicated in the etiology of type 2 diabetes. Various proteins/lipases of Mtb are involved in the activation of immune cells and thus involved in triggering inflammatory responses in the host. Modulation of adipose tissue signaling during tuberculosis may be one of the reasons of metabolic disorder and pre-diabetic conditions during tuberculosis. Adipose tissue infection by Mtb causes tissue inflammation, immune cell infiltration and modulation of adipokine secretion which altogether may be involved in generation of pre-diabetic conditions. Loss of adipose tissue due to increased lipolysis during tuberculosis leads to release of free fatty acids which in turn disrupts insulin signaling pathway in skeletal muscles and can cause systemic insulin resistance. Various Mtb proteins are responsible for host metabolic perturbation and modulation of lipid metabolism. Again, reduction in circulating amino acid levels like histidine, tryptophan, methionine and glutamine may diminish host capacity to resists pre-diabetic changes. A detail study on the molecular mechanisms by which M. tuberculosis disrupts host glucose homeostasis and understanding the signaling cascades is important to develop appropriate therapeutics to tackle hyperglycemic condition during TB.
3 Impact of diabetes on the susceptibility to tuberculosis
As a chronic condition diabetes compromises the immune system which reduce the host’s ability to fight infections. It has been found that, diabetes can cause impairment of cytokine production, inhibit leukocyte recruitment, dysfunction of immune cells like neutrophil, macrophages, and natural killer cells. In addition to dysfunction of the cellular immunity, it can affect complement effector system and antibody production (Berbudi et al., 2020). In fact diabetes increases susceptibility to various infections like HIV (Grinspoon, 2009), fungal infections (Lao et al., 2020), mycobacterium (Kumar and Babu 2017; Navid et al., 2020) and recently COVID-19 (Erener, 2020). Type 2 diabetes is considered to be a major risk factor for increased susceptibility to TB infection and reactivation (Baker et al., 2011; Yorke et al., 2017; Mukhtar and Butt, 2018; Ayelign et al., 2019; Alim et al., 2020). Diabetic individuals were found to be three times more susceptible to TB (Jeon and Murray, 2008) and also have higher risk of death during TB treatment (Gautam et al., 2021), poor TB treatment outcomes and higher risk of developing latent TB (Restrepo, 2016; vanCrevel and Critchley, 2021). Conditions which complicate TB pathology, outcome of treatment and mortality are age, poorer immune activity, inflammation, hyperglycemia, liver and chronic kidney disease which are often associated with diabetes (Alim et al., 2020; Kaur et al., 2021).
The major immune mechanisms by which diabetic patients become more susceptible to TB include but not limited to defects in bacterial recognition, reduced phagocytic activity, the slow migration rate of macrophages and other antigen-presenting cells, secretion of chemokines/cytokines and impaired T cell response that ultimately results in compromised immune responses causing increased Mtb load and disease pathogenicity in various organs like lungs and liver (Vallerskog et al., 2010; Kumar et al., 2017b; Alim et al., 2020). It has been found that the function of neutrophils, macrophages, dendritic cells, NK cells and other components of innate immunity is drastically compromised by metabolic alterations (Martinez and Kornfeld, 2014). Alveolar macrophages from chronically hyperglycemic mice were found to have impaired recognition of M. tuberculosis due to reduced expression of CD14 and MARCO which are involved in recognition of mycobacterial cell wall components (Martinez et al., 2016). In addition, peripheral monocytes from diabetic patients were found to have reduced capacity to bind or engulf Mtb bacilli compared to those from euglycemic controls due to modifications in the complement pathway of opsonization (Gomez et al., 2013).
Among the phagocytic cells predominantly, the neutrophils and macrophages are primarily responsible for the induction of inflammatory response during tuberculosis and have a detrimental effect on TB-DM pathogenesis (Das and Mukhopadhyay, 2011; Mishra et al., 2017; Borkute et al., 2021). Neutrophils harboring M. tuberculosis are abundant in inflammatory lesions and associated with lung pathology and TB disease severity (Muefong and Sutherland, 2020). Neutrophil counts are higher during TB infection and TB-DM further increases neutrophil count (Prada-Medina et al., 2017). Higher neutrophil leukocyte ratio is a potential marker of inflammation and can cause TB disease severity (Abakay et al., 2015), insulin resistance and type 2 diabetes (Guo et al., 2015; Lou et al., 2015). Tissue-specific inflammation caused by Mtb infection can also cause modulation of signaling mechanisms and metabolic abnormality. Mtb infection in adipose tissue causes mTOR/Akt-mediated inflammation and immune cell infiltration into adipose tissues, which is responsible for adipocyte hypertrophy, increased insulin sensitivity and may result in systemic glucose tolerance (Martinez et al., 2019). Interestingly, neutrophils in hyperglycemic subjects had reduced chemotaxis (Tater et al., 1987), phagocytic defect and reduced microbicidal activity (Chanchamroen et al., 2009).
It is apparent that a proper Th1-biased adaptive immune response is the major determinant of outcomes in human TB and in animal models of TB. Delayed priming of cell-mediated immunity appears to be one of the major reasons for higher susceptibility to tuberculosis in hyperglycemic mice (Vallerskog et al., 2010). IFNγ produced by Th1 cells is critical for protective immune response of the host while IL-2 is essential for the development and proliferation of Th1 and CD8+ cells. In streptozotocin-induced diabetes mellitus mice model, expression of Th1 cytokines and inducible nitric oxide synthase was found to be lower upon infection with Mtb (Yamashiro et al., 2005). However, in diabetic individuals, IFNγ secretion by immune cells was found to be not uniform. Stimulation of immune cells with mycobacterial antigens, either no difference (Stalenhoef et al., 2008) or lower (Meenakshi et al., 2016) or higher (Gan et al., 2014) secretion of IFN-γ was observed in DM patients compared to healthy controls. In other studies, hyperglycemia was found to be associated with suppression of innate and adaptive immune responses in TB-DM patients as absolute numbers of total T and B lymphocytes, CD8+ T lymphocytes, and NK cells were found to be lower (Wei et al., 2022). Experiments from mice model of infection suggest that an impaired innate response to initial infection with a resulting delay in the adaptive immune effector response is the key mechanism of susceptibility to tuberculosis in diabetes (Martinez and Kornfeld, 2014).
Diabetics with latent TB infection (LTBi) had increased inflammatory responses indicating that diabetic condition influences immune signaling to mycobacterial antigens (Ssekamatte et al., 2021; Masood et al., 2022). In a diet-induced type 2 diabetes mice model (that reflects the features of human T2D), a higher mortality rate, increased lung and liver bacilli burden and higher inflammatory lesions were observed as compared to non-diabetic control mice (Alim et al., 2017; Alim et al., 2019). These mice showed impaired macrophage anti-mycobactericidal function, higher inflammatory lesions and impaired cytokine kinetics (TNF-α, MCP-1, IL-12, IFN-γ) (Alim et al., 2017). In another study, streptozotocin-induced DM had delayed priming of adaptive immune response leading to impaired TB defence in mice (Vallerskog et al., 2010). Even latent TB patients with pre-diabetic conditions have diminished frequencies of antigen–specific Th1/Tc1 and Th17/Tc17 cells, indicating that pre-diabetic condition is associated with alterations of the immune response with compromised CD4+ and CD8+T cell function in latent TB cases (Kumar et al., 2017a). The reduction of innate and adaptive immunity in diabetic patients causes the severity of TB disease and might be involved in adverse effects of TB treatment outcome, increased morbidity and mortality during TB-DM (Workneh et al., 2016; Prada-Medina et al., 2017). Higher levels of pro-inflammatory cytokines were observed in TB-DM patients compared to non-diabetic patients before and throughout the anti-tuberculosis therapy (Kumar et al., 2019). TB-DM comorbidity is attributed to excessive and prolonged inflammation and organ damage and morbidity/mortality in pulmonary TB largely reflects the consequences of immune-mediated lung damage (Ravimohan et al., 2018). Hyperglycaemic patients are likely to develop cavitary lung lesions and poor treatment outcomes with higher post-treatment mortality (Williams et al., 2022). In fact, diabetic patients were found to develop more frequent lung cavities and parenchymal lesions upon chest X-ray (Chiang et al., 2014). diabetic patients with poor glycemic control are likely to be presented with atypical findings upon chest X-ray and thoracic CT scans, such as advanced extensive lesions (p < 0.001), more cavities (p < 0.001) and all-lobe involvement (Huang et al., 2017). Hyperglycemia in DM patients suggested to be a risk factor for pulmonary cavity formation and lobe lesions in patients with TB-DM which adds to TB pathology (Wei et al., 2022). Chronic renal failure is another risk factor for the infection progression of tuberculosis and chronic renal failure and dialysis increase the risk of TB infection by 6.9 to 52.5-fold compared to the general population (Hussein et al., 2003). Thus, both type 1 and type 2 diabetes decrease immune response against tuberculosis increasing the chance of organ pathology and disease susceptibility with complications of anti-TB treatment and death in TB.
4 Therapeutic management
Searching for strategies to prevent diabetic conditions is of utmost importance. Various factors, like pro-inflammatory cytokines, inflammation, reactive oxygen species, glyco/lipoxidation end products are shown to be involved in the pathogenesis of diabetic kidney disease, diabetic retinopathy, and on the progression of atherosclerosis and diabetes-associated non-alcoholic fatty liver disease (NAFLD). Mtb can act in a pleiotropic manner affecting adipose tissue signaling, inflammation, host metabolism (glucose and lipid) causing hyperglycaemia and insulin resistance that may lead to diabetes. Thus, strategies need to be devised to control/manipulate such factors during infection with Mtb to regulate diabetes and associated disorders in human. It has been shown that drugs used to treat tuberculosis can worsen the glycaemic control in diabetic patients. Overlapping toxicities should be considered when co-managing tuberculosis and diabetes. It is suggested that pyridoxine should be given with isoniazid during tuberculosis treatment in diabetic patients considering the risk of peripheral neuropathy (American Thoracic Society, CDC, Infectious Diseases Society of America, 2003). Treatment with rifampicin probably causes hyperglycaemia via interactions with oral hypoglycaemic drugs (Atkin et al., 1992; Niemi et al., 2001). Rifampicin causes early-phase hyperglycaemia with associated hyperinsulinaemia even in non-diabetic patients (Takasu et al., 1982; Waterhouse et al., 2005). Therefore, careful attention needs to be opted in delivering the anti-tuberculosis drugs in managing hyperglycaemia and diabetes. Also, therapeutics may be designed tox inhibit excess inflammation during tuberculosis. Since expression of MCP-1 was shown to be correlated with appearance of insulin resistance, management of MCP-1 expression during TB could serve as an important strategy to control type 2 diabetes. During infection, Mtb shifts the carbon source from glucose to fatty acids and utilizes glyoxylate cycle (Mukhopadhyay et al., 2012). Expression of the gating enzyme of glyoxylate cycle, isocitrate lyase (ICL) is upregulated during infection, and is important for survival during the persistent phase of infection. Thus, blocking of ICL could be important to tackle the Mtb infection and Mtb-associated systemic metabolic complications (Erol, 2008). One of the possible mechanisms to counter the manipulating effect of Mtb is to target its ability to modify host metabolism (glucose and lipid). It is thus important to target the secretory/effector proteins/molecules of Mtb (like ESAT-6 and lipases) that cause metabolic perturbation. Also, management of inflammation during TB could be another therapeutic measurement to control hyperglycaemia and type 2 diabetes. An interesting study indicates that binding of Mtb proteins specifically to 16~20 TLR2 LRR domain can trigger NF-κB signaling and TNF-α production in macrophages indicating the importance of such signaling in the regulation of pro-inflammation response which may lead to insulin resistance and type 2 diabetes (Bhat et al., 2012). Thus, the site of interaction on TLR2 can dictate the downstream signaling events leading to activation of NF-κB and pro-inflammation, which can be manipulated in the regulation of inflammatory signaling during TB diseases by designing appropriate drugs to specifically block TLR2 LRR 16~20-triggered signaling cascades. Another study indicates that cellular localization of Mtbhsp60 post interaction with TLRs can dictate the type of polarization in the innate immune responses in macrophages (Parveen et al., 2013). The results indicate that NF-κB and TNF-α activation by Mtbhsp60 was restricted predominantly to TLR4-triggered signaling. This information is useful in devising strategies to manipulate macrophage innate responses and block the excess pro-inflammatory signaling during TB which may be useful to tackle type 2 diabetes. Thus, therapeutic strategies targeting inflammation may be important for designing of effective treatment of diabetic complications during TB. Interestingly, a recent study highlights the protective efficacy of ESX-1-expressing BCG strains against Mtb infection in a diet-induced murine model of type 2 diabetes which is due to increased numbers and activation of antigen presenting cells in the lungs of diabetic mice (Sathkumara et al., 2020). Important strategies to stop development of TB might include, early diagnosis and early treatment adaptation, targeted vaccination programmes, screening for latent TB and diabetes among TB patients as well as improved diabetes management and prevention.
5 Conclusion and future perspective
TB-DM co-incidence is a major concern among tuberculosis patients as it complicates treatment course and disease outcome drastically. Various studies support that TB can influence the pathogenesis and incidence of diabetes (Mugusi et al., 1990; Magee et al., 2018). Occurrence of hyperglycaemia, insulin resistance and metabolic alterations during tuberculosis suggests the involvement of Mtb factors/proteins in development of pre-diabetic/diabetic conditions. Although various studies support that changes in immune responses/signaling cascades and host physiology during Mtb infection can induce hyperglycaemia that can stimulate pre-diabetic conditions, many of the important questions regarding host factors and signaling cascades that can influence occurrence of diabetes in TB patients are yet to be answered. It is very important to identify what Mtb factor is involved in development of hyperglycaemia and pre-diabetic/diabetic conditions which is crucial for the design of appropriate therapeutics to prevent this patho-physiological disorder during TB. Another important issue is the status of DM prevalence with respect to MDR/XDR TB which has to be seriously investigated. Also, studies need to be focused to understand the influence of dormancy/latency on TB-DM as diabetes and latent TB can be considered as predisposing factors for TB progression. Considering, the increasing cases of TB-DM, and the facts that TB-DM can enhance DM-linked complication pathways compared to DM alone (Prada-Medina et al., 2017), and also TB-DM co-occurrence can cause adverse effect on TB treatment and outcome of disease, the regular screening of TB patients for diabetes is important. Further, the host-directed therapy approach is important for TB-DM treatment to control immune-modulation and metabolic alterations that occurs during tuberculosis.
Author contributions
MB — Conceptualization, Preparation of first draft and revision. PD — Writing and review. SG — Manuscript writing and editing. SM — Conceptualization, writing, review and finalization of the manuscript. All authors contributed to the article and approved the submitted version.
Funding
The laboratory of SM is supported by the grant from the Department of Biotechnology (DBT), Govt. of India (BT/PR35722/BRB/10/1837/2019), Department of Science and Technology-Science and Engineering Research Board (DSTSERB), Govt. of India (JCB/2021/000035, CRG/2019/000239), Council of Scientific and Industrial Research (CSIR), Govt. of India (27(0364)/20/EMRII), Indian Council of Medical Research (ICMR), Govt of India (2021-10087/GTGE/ADHOC-BMS) and a core grant from CDFD by DBT. MKB and PD are supported by the fellowships from Council of Scientific and Industrial Research (CSIR), Govt. of India and DBT respectively.
Acknowledgments
The author thanks Dr Sandeep Kumar Kotturu for discussion.
Conflict of interest
The authors declare that the research was conducted in the absence of any commercial or financial relationships that could be construed as a potential conflict of interest.
Publisher’s note
All claims expressed in this article are solely those of the authors and do not necessarily represent those of their affiliated organizations, or those of the publisher, the editors and the reviewers. Any product that may be evaluated in this article, or claim that may be made by its manufacturer, is not guaranteed or endorsed by the publisher.
Abbreviations
TB, Tuberculosis; Mtb, Mycobacterium tuberculosis; HIV, human immunodeficiency virus; DM, Type 2 diabetes mellitus; TB-DM, Tuberculosis and diabetes; WHO, World Health organization; MDR, Multidrug-resistance; IR, Insulin resistance; LDL, Low-density lipoprotein; HDL, High-density lipoprotein; IGT, Impaired glucose tolerance; PI3K, Phosphoinositide 3-kinase; HbA1C, Hemoglobin A1C; IFN-γ, Interfero-gamma; TNF-α,Tumor necrosis factor-α; TLR, Toll like receptor; NF-Kb, Nuclear factor κ B; HFD, High fat diet; T2D, Type 2 diabetes; IL, Interleukin; MCP-1, Monocyte chemoattractant protein-1; PPAR-g, Peroxisome proliferator-activated receptor-γ; GLUT4, Glucose transporter type 4; NK, Natural killer cells; TNFR, Tumor necrosis factor receptor; Camp, Cyclic adenosine monophosphate; ROS, Reactive oxygen species; NADPH, Nicotinamide adenine dinucleotide phosphate; PRRs, Pattern recognition receptors; HIF-1a, Hypoxia-inducible factor-1alpha; COVID-19, Coronavirus disease; ACSL1, Acyl-CoA Synthetase long-chain family member 1; Th1, T-helper 1; NAFLD, Non-alcoholic fatty liver disease; CT scan, Computerized tomography scan.
References
Abakay., O., Abakay, A., Sen, H. S., Tanrikulu, A. C. (2015). The relationship between inflammatory marker levels and pulmonary tuberculosis severity. Inflammation 38, 691–696. doi: 10.1007/s10753-014-9978-y
Agarwal, P., Gordon, S., Martinez, F. O. (2021). Foam cell macrophages in tuberculosis. Front. Immunol. 12, 775326. doi: 10.3389/fimmu.2021.775326
Ahmad, R., Al-Mass, A., Atizado, V., Al-Hubail, A., Al-Ghimlas, F., Al-Arouj, M., et al. (2012). Elevated expression of the toll-like receptors 2 and 4 in obese individuals: its significance for obesity-induced inflammation. J. Inflamm. (Lond) 9, 48. doi: 10.1186/1476-9255-9-48
Akira, S., Takeda, K. (2004). Toll-like receptor signalling. Nat. Rev. Immunol. 4, 499–511. doi: 10.1038/nri1391
Alim, M. A., Kupz, A., Sikder, S., Rush, C., Govan, B., Ketheesan, N. (2020). Increased susceptibility to Mycobacterium tuberculosis infection in a diet-induced murine model of type 2 diabetes. Microbes Infect. 22, 303–311. doi: 10.1016/j.micinf.2020.03.004
Alim, M. A., Sikder, S., Bridson, T. L., Rush, C. M., Govan, B. L., Ketheesan, N. (2017). Anti-mycobacterial function of macrophages is impaired in a diet induced model of type 2 diabetes. Tuberculosis (Edinb). 102, 47–54. doi: 10.1016/j.tube.2016.12.002
Alim, M. A., Sikder, S., Sathkumara, H., Kupz, A., Rush, C. M., Govan, B. L., et al. (2019). Dysregulation of key cytokines may contribute to increased susceptibility of diabetic mice to Mycobacterium bovis BCG infection. Tuberculosis (Edinb). 115, 113–120. doi: 10.1016/j.tube.2019.02.005
American Thoracic Society, CDC, Infectious Diseases Society of America (2003). Treatment of tuberculosis. MMWR Recomm. Rep. 52, 1–77.
Appay, V., Rowland-Jones, S. L. (2001). RANTES: a versatile and controversial chemokine. Trends Immunol. 22, 83–87. doi: 10.1016/s1471-4906(00)01812-3
Asensio, C., Cettour-Rose, P., Theander-Carrillo, C., Rohner-Jeanrenaud, F., Muzzin, P. (2004). Changes in glycemia by leptin administration or high- fat feeding in rodent models of obesity/type 2 diabetes suggest a link between resistin expression and control of glucose homeostasis. Endocrinology 145, 2206–2213. doi: 10.1210/en.2003-1679
Atkin, S. H., Jaker, M. A., Beaty, P., Quadrel, M. A., Cuffie, C., Soto-Greene, M. L. (1992). Oral labetalol versus oral clonidine in the emergency treatment of severe hypertension. Am. J. Med. Sci. 303, 9–15. doi: 10.1097/00000441-199201000-00004
Atkin, S. L., Masson, E. A., Bodmer, C. W., Walker, B. A., White, M. C. (1993). Increased insulin requirement in a patient with type 1 diabetes on rifampicin. Diabetes Med. 10, 392. doi: 10.1111/j.1464-5491.1993.tb00086.x
Atlas IDFD (2021) International diabetes federation. Available at: https://diabetesatlas.org/atlas/tenth-edition/.
Ayelign, B., Negash, M., Genetu, M., Wondmagegn, T., Shibabaw, T. (2019). Immunological impacts of diabetes on the susceptibility of Mycobacterium tuberculosis. J. Immunol. Res. 2019, 6196532. doi: 10.1155/2019/6196532
Ayyappan, J. P., Ganapathi, U., Lizardo, K., Vinnard, C., Subbian, S., Perlin, D. S., et al. (2019). Adipose tissue regulates pulmonary pathology during TB infection. MBio 10, e02771–e02718. doi: 10.1128/mBio.02771-18
Badin, P. M., Louche, K., Mairal, A., Liebisch, G., Schmitz, G., Rustan, A. C., et al. (2011). Altered skeletal muscle lipase expression and activity contribute to insulin resistance in humans. Diabetes 60, 1734–1742. doi: 10.2337/db10-1364
Baker, M. A., Harries, A. D., Jeon, C. Y., Hart, J. E., Kapur, A., Lonnroth, K., et al. (2011). The impact of diabetes on tuberculosis treatment outcomes: a systematic review. BMC Med. 9, 81. doi: 10.1186/1741-7015-9-81
Banerjee, J., Mishra, N., Damle, G., Dhas, Y. (2019). Beyond LDL-c: the importance of serum oxidized LDL in predicting risk for type 2 diabetes in the middle-aged Asian indians. Diabetes Metab. Syndr. 13, 206–213. doi: 10.1016/j.dsx.2018.08.036
Beigier-Bompadre, M., Montagna, G. N., Kühl, A. A., Lozza, L., Weiner, J., Kupz, A., et al. (2017). Mycobacterium tuberculosis infection modulates adipose tissue biology. PloS Pathog. 13, e1006676. doi: 10.1371/journal.ppat.1006676
Berbudi, A., Rahmadika, N., Tjahjadi, A. I., Ruslami, R. (2020). Type 2 diabetes and its impact on the immune system. Curr. Diabetes Rev. 16, 442–449. doi: 10.2174/1573399815666191024085838
Bhat, K. H., Chaitanya, C. K., Parveen, N., Varman, R., Ghosh, S., Mukhopadhyay, S. (2012). Proline-proline-glutamic acid (PPE) protein Rv1168c of Mycobacterium tuberculosis augments transcription from HIV-1 long terminal repeat promoter. J. Biol. Chem. 287, 16930–16946. doi: 10.1074/jbc.M111.327825
Billig, S., Schneefeld, M., Huber, C., Grass, G. A., Eisenreich, W., Range, F. C. (2017). Lactate oxidation facilitates growth of Mycobacterium tuberculosis in human macrophages. Sci. Rep. 7, 6484. doi: 10.1038/s41598-017-05916-7
Biranu, E., Wolde, M., Negesso, A. E., Sisay, M. M., Tola, H. H. (2021). Lipid profile, abnormality of serum glucose levels and their associated factors in multidrug-resistant tuberculosis patients. Glob. J. Obes. Diabetes Metab. Syndr. 8, 018–028. doi: 10.17352/2455-8583.000053
Boden, G. (2003). Effects of free fatty acids (FFA) on glucose metabolism: significance for insulin resistance and type 2 diabetes. Exp. Clin. Endocrinol. Diabetes 111, 121–124. doi: 10.1055/s-2003-39781
Boden, G., Shulman, G. I. (2002). Free fatty acids in obesity and type 2 diabetes: defining their role in the development of insulin resistance and β-cell dysfunction. Eur. J. Clin. Invest. 32, 14–23. doi: 10.1046/j.1365-2362.32.s3.3.x
Borah, K., Girardi, D. V., Mendum, T. A., Santos, M., Beste, D. J. V., Lara, A., et al. (2019). Intracellular Mycobacterium leprae utilizes host glucose as a carbon source in schwann cells. MBio 10, 1–9. doi: 10.1128/mBio.02351-19
Borkute, R. R., Woelke, S., Pei, G., Dorhoi, A. (2021). Neutrophils in tuberculosis: cell biology, cellular networking and multitasking in host defense. Int. J.Mol. Sci. 22, 4801. doi: 10.3390/ijms22094801
Burhans, M. S., Hagman, D. K., Kuzma, J. N., Schmidt, K. A., Kratz, M. (2019). Contribution of adipose tissue inflammation to the development of type 2 diabetes mellitus. Compr. Physiol. 9, 1–58. doi: 10.1002/cphy.c170040
Cambier, C. J., Takaki, K. K., Larson, R. P., Hernandez, R. E., Tobin, D. M., Urdahl, K. B., et al. (2014). Mycobacteria manipulate macrophage recruitment through coordinated use of membrane lipids. Nature 505, 218–222. doi: 10.1038/nature12799
Casqueiro, J., Casqueiro, J., Alves, C. (2012). Infections in patients with diabetes mellitus: a review of pathogenesis. Indian J. Endocrinol. Metab. 16, 27–36. doi: 10.4103/2230-8210.94253
Chanchamroen, S., Kewcharoenwong, C., Susaengrat, W., Ato, M., Lertmemongkolchai, G. (2009). Human polymorphonuclear neutrophil responses to Burkholderia pseudomallei in healthy and diabetic subjects. Infect. Immun. 77, 456–463. doi: 10.1128/IAI.00503-08
Chang, S. W., Pan, W. S., Lozano, B. D., Oleyda, B. L., Solano, M. A., Tuero, I., et al. (2013). Gut hormones, appetite suppression and cachexia in patients with pulmonary TB. PloS One 8, e54564. doi: 10.1371/journal.pone.0054564
Chen, M., Divangahi, M., Gan, H., Shin, D. S., Hong, S., Lee, D. M., et al. (2008). Lipid mediators in innate immunity against tuberculosis: opposing roles of PGE2 and LXA4 in the induction of macrophage death. J. Exp. Med. 205, 2791–2801. doi: 10.1084/jem.20080767
Chen, N., Zhou, L., Zhang, Z., Xu, J., Wan, Z., Qin, L. (2014). Resistin induces lipolysis and suppresses adiponectin secretion in cultured human visceral adipose tissue. Regul. Pept. 194-195, 49–54. doi: 10.1016/j.regpep.2014.10.001
Chiang, C. Y., Lee, J. J., Chien, S. T., Enarson, D. A., Chang, Y. C., Chen, Y. T., et al. (2014). Glycemic control and radiographic manifestations of tuberculosis in diabetic patients. PloS One 9, e93397. doi: 10.1371/journal.pone.0093397
Chistiakov, D. A., Melnichenko, A. A., Myasoedova, V. A., Grechko, A. V., Orekhov, A. N. (2017). Mechanisms of foam cell formation in atherosclerosis. J. Mol. Med. 95, 1153–1165. doi: 10.1007/s00109-017-1575-8
Chou, S. Y., Ajoy, R., Changou, C. A., Hsieh, Y. T., Wang, Y. K., Hoffer, B. (2016). CCL5/RANTES contributes to hypothalamic insulin signaling for systemic insulin responsiveness through CCR5. Sci. Rep. 6, 37659. doi: 10.1038/srep37659
Corcoran, S. E., O'Neill, L. A. (2016). HIF1α and metabolic reprogramming in inflammation. J. Clin. Investig. 126, 3699–3707. doi: 10.1172/JCI84431
Creely, S. J., McTernan, P. G., Kusminski, C. M., Fisher, M., Da Silva, N. F., Khanolkar, M., et al. (2007). Lipopolysaccharide activates an innate immune system response in human adipose tissue in obesity and type 2 diabetes. Am. J. Physiol. Endocrinol. Metab. 292, E740–E747. doi: 10.1152/ajpendo.00302.2006
Daryabor, G., Atashzar, M. R., Kabelitz, D., Meri, S., Kalantar, K. (2020). The effects of type 2 diabetes mellitus on organ metabolism and the immune system. Front. Immunol. 11. doi: 10.3389/fimmu.2020.01582
Das, A., Mukhopadhyay, S. (2011). The evil axis of obesity, inflammation and type-2 diabetes. Endocr. Metab. Immune Disord. Drug Targets 11, 23–31. doi: 10.2174/187153011794982086
Dasu, M. R., Devaraj, S., Park, S., Jialal, I. (2010). Increased toll-like receptor (TLR) activation and TLR ligands in recently diagnosed type 2 diabetic subjects. Diabetes Care 33, 861–868. doi: 10.2337/dc09-1799
Dedieu, L., Serveau-Avesque, C., Kremer, L., Canaan, S. (2013). Mycobacterial lipolytic enzymes: a gold mine for tuberculosis research. Biochimie 95, 66–73. doi: 10.1016/j.biochi.2012.07.008
Delarue, J., Magnan, C. (2007). Free fatty acids and insulin resistance. Curr. Opin. Clin. Nutr. Metab. Care 10, 142–148. doi: 10.1097/MCO.0b013e328042ba90
Deng, C., Wang, X., Liao, Y. (2016). Current recommendations on managing tuberculosis patients with diabetes & its epidemiology. Microb. Pathog. 92, 43–45. doi: 10.1016/j.micpath.2015.12.005
Dhouib, R., Laval, F., Carrière, F., Daffé, M., Canaan, S. (2010). A monoacylglycerol lipase from Mycobacterium smegmatis involved in bacterial cell interaction. J. Bacteriol. 192, 4776–4785. doi: 10.1128/JB.00261-10
Ding, Y., Raterink, R. J., Marín-Juez, R., Veneman, W. J., Egbers, K., van den Eeden, S., et al. (2020). Tuberculosis causes highly conserved metabolic changes in human patients, mycobacteria-infected mice and zebrafish larvae. Sci. Rep. 10, 11635. doi: 10.1038/s41598-020-68443-y
Dolasia, K., Bisht, M. K., Pradhan, G., Udgata, A., Mukhopadhyay, S. (2018). TLRs/NLRs: shaping the landscape of host immunity. Int. Rev. Immunol. 37, 3–19. doi: 10.1080/08830185.2017.1397656
Dong, J., Yang, S., Zhuang, Q., Sun, J., Wei, P., Zhao, X. (2021). The associations of lipid profiles with cardiovascular diseases and death in a 10-year prospective cohort study. Front. Cardiovasc. Med. 8. doi: 10.3389/fcvm.2021.745539
Dorrington, M. G., Fraser, I. D. C. (2019). NF-κB signaling in macrophages: dynamics, crosstalk, and signal integration. Front. Immunol. 10. doi: 10.3389/fimmu.2019.00705
Ephraim, R. K. D., Baah, S. K., Sakyi, S. A., Darkwah, K. O., Abaka-Yawson, A. (2021). Hyperglycaemia in newly diagnosed pulmonary tuberculosis patients: a cross-sectional study of the agona district hospital. Ann. Med. Lab. Sci. 1, 50–58. doi: 10.51374/annalsmls.2021.1.2.0042
Erener, S. (2020). Diabetes, infection risk and COVID-19. Mol. Metab. 39, 101044. doi: 10.1016/j.molmet.2020.101044
Erol, A. (2008). Visceral adipose tissue specific persistence of Mycobacterium tuberculosis may be reason for the metabolic syndrome. Med. Hypotheses 71, 222–228. doi: 10.1016/j.mehy.2008.03.028
Evangelista, M. S. N., Maia, R., Toledo, J. P., Abreu, G. R., Barreira, D. (2020). Tuberculosis associated with diabetes mellitus by age group in Brazil: a retrospective cohort study 2007–2014. Braz. J. Infect. Dis. 24, 130–136. doi: 10.1016/j.bjid.2020.03.005
Ferrucci, L., Fabbri, E. (2018). Inflammageing: chronic inflammation in ageing, cardiovascular disease, and frailty. Nat. Rev. Cardiol. 15, 505–522. doi: 10.1038/s41569-018-0064-2
Gan, S. H., KhinMar, K. W., Barkham, T. M., Koh, C. K., Shen, L., Wang, Y. T., et al. (2014). Interferon-γ responses to Mycobacterium tuberculosis-specific antigens in diabetes mellitus. Eur. Respir. J. 44, 805–808. doi: 10.1183/09031936.00226613
Gautam, S., Shrestha, N., Mahato, S., Nguyen, T. P. A., Mishra, S. R. (2021). Diabetes among tuberculosis patients and its impact on tuberculosis treatment in south Asia: a systematic review and meta−analysis. Sci. Rep. 11, 2113. doi: 10.1038/s41598-021-81057-2
German, J. P., Wisse, B. E., Thaler, J. P., Oh-I, S., Sarruf, D. A., Ogimoto, K., et al. (2010). Leptin deficiency causes insulin resistance induced by uncontrolled diabetes. Diabetes 59, 1626–1634. doi: 10.2337/db09-1918
Gharibeh, M. Y., Al Tawallbeh, G. M., Abboud, M. M., Radaideh, A., Alhader, A. A., Khabour, O. F. (2010). Correlation of plasma resistin with obesity and insulin resistance in type 2 diabetic patients. Diabetes Metab. 36, 443–449. doi: 10.1016/j.diabet.2010.05.003
Gharravi, A. M., Jafar, A., Ebrahimi, M., Mahmodi, A., Pourhashemi, E., Haseli, N., et al. (2018). Current status of stem cell therapy, scaffolds for the treatment of diabetes mellitus. Diabetes Metab. Syndr. 12, 1133–1139. doi: 10.1016/j.dsx.2018.06.021
Gomez, D. I., Twahirwa, M., Schlesinger, L. S., Restrepo, B. I. (2013). Reduced Mycobacterium tuberculosis association with monocytes from diabetes patients that have poor glucose control. Tuberculosis 93, 192–197. doi: 10.1016/j.tube.2012.10.003
Green, A., Rumberger, J. M., Stuart, C. A., Ruhoff, M. S. (2004). Stimulation of lipolysis by tumor necrosis factor-α in 3T3-L1 adipocytes is glucose dependent: implications for long-term regulation of lipolysis. Diabetes 53, 74–81. doi: 10.2337/diabetes.53.1.74
Grininger, C., Leypold, M., Aschauer, P., Pavkov-Keller, T., Riegler-Berket, L., Breinbauer, R., et al. (2021). Structural changes in the cap of Rv0183/mtbMGL modulate the shape of the binding pocket. Biomolecules 11, 1299. doi: 10.3390/biom11091299
Grinspoon, S. (2009). Diabetes mellitus, cardiovascular risk, and HIV disease. Circulation. 119, 770–772. doi: 10.1161/CIRCULATIONAHA.108.835710
Guerrini, V., Prideaux, B., Blanc, L., Bruiners, N., Arrigucci, R., Singh, S., et al. (2018). Storage lipid studies in tuberculosis reveal that foam cell biogenesis is disease-specific. PloS Pathog. 14, e1007223. doi: 10.1371/journal.ppat.1007223
Guilherme, A., Virbasius, J. V., Puri, V., Czech, M. P. (2008). Adipocyte dysfunctions linking obesity to insulin resistance and type 2 diabetes. Nat. Rev. Mol. Cell Bio. 9, 367–377. doi: 10.1038/nrm2391
Guo, X., Zhang, S., Zhang, Q., Liu, L., Wu, H., Du, H. (2015). Neutrophil:lymphocyte ratio is positively related to type 2 diabetes in a large-scale adult population: a tianjin chronic low-grade systemic inflammation and health cohort study, eur. J. Endocrinol. 173, 217–225. doi: 10.1530/EJE-15-0176
He, J., Fan, Y., Shen, D., Yu, M., Shi, L., Ding, S., et al. (2020). Characterization of cytokine profile to distinguish latent tuberculosis from active tuberculosis and healthy controls. Cytokine 135, 155218. doi: 10.1016/j.cyto.2020.155218
Heldwein, K. A., Fenton, M. J. (2002). The role of toll-like receptors in immunity against mycobacterial infection. Microbes Infect. 4, 937–944. doi: 10.1016/s1286-4579(02)01611-8
Hotamisligil, G. S. (2017). Inflammation, metaflammation and immunometabolic disorders. Nature 542, 177–185. doi: 10.1038/nature21363
Howard, N. C., Khader, S. A. (2020). Immunometabolism during Mycobacterium tuberculosis infection. Trends Microbiol. 28, 832–850. doi: 10.1016/j.tim.2020.04.010
Huaman, M. A., Henson, D., Ticona, E., Sterling, T. R., Garvy, B. A. (2015). Tuberculosis and cardiovascular disease: linking the epidemics. Trop. Dis. Travel Med. Vaccines 1, 10. doi: 10.1186/s40794-015-0014-5
Huang, L. K., Wang, H. H., Lai, Y. C., Chang, S. C. (2017). The impact of glycemic status on radiological manifestations of pulmonary tuberculosis in diabetic patients. PloS One 12, e0179750. doi: 10.1371/journal.pone.0179750
Hussein, M. M., Mooij, J. M., Roujouleh, H. (2003). Tuberculosis and chronic renal disease. Semin. Dial. 16, 38–44. doi: 10.1046/j.1525-139x.2003.03010.x
Jeon, C. Y., Harries, A. D., Baker, M. A., Hart, J. E., Kapur, A., Lönnroth, K., et al. (2010). Bi-directional screening for tuberculosis and diabetes: a systematic review. Trop. Med. Int. Health 15, 1300–1314. doi: 10.1111/j.1365-3156.2010.02632.x
Jeon, C. Y., Murray, M. B. (2008). Diabetes mellitus increases the risk of active tuberculosis: a systematic review of 13 observational studies. PloS Med. 5, e152. doi: 10.1371/journal.pmed.0050152
Jung, H. S., Shimizu-Albergine, M., Shen, X., Kramer, F., Shao, D., Vivekanandan-Giri, A., et al. (2020). TNF-α induces acyl-CoA synthetase 3 to promote lipid droplet formation in human endothelial cells. J. Lipid Res. 61, 33–44. doi: 10.1194/jlr.RA119000256
Kanagawa, H., Niki, Y., Kobayashi, T., Sato, Y., Katsuyama, E., Fujie, A., et al. (2015). Mycobacterium tuberculosis promotes arthritis development through toll-like receptor 2. J. Bone Miner. Metab. 33, 135–141. doi: 10.1007/s00774-014-0575-9
Kaufmann, S. H., Dorhoi, A. (2013). Inflammation in tuberculosis: interactions, imbalances and interventions. Curr. Opin. Immunol. 25, 441–449. doi: 10.1016/j.coi.2013.05.005
Kaur, R., Egli, T., Paynter, J., Murphy, R., Perumal, L., Lee, A., et al. (2021). Tuberculosis and diabetes: increased hospitalisations and mortality associated with renal impairment. Intern. Med. J. doi: 10.1111/imj.15668
Kaur, G., Saini, V., Kumari, B., Kaur, J., Kaur, J. (2017). Characterization of an extracellular protein, Rv1076 from M. tuberculosis with a potential role in humoral response. Int. J. Biol. Macromol. 101, 621–629. doi: 10.1016/j.ijbiomac.2017.03.096
Kelesidis, T., Kelesidis, I., Chou, S., Mantzoros, C. S. (2010). Narrative review: the role of leptin in human physiology: emerging clinical applications. Ann. Intern. Med. 152, 93–100. doi: 10.7326/0003-4819-152-2-201001190-00008
Keophiphath, M., Rouault, C., Divoux, A., Clément, K., Lacasa, D. (2010). CCL5 promotes macrophage recruitment and survival in human adipose tissue. Arterioscler. Thromb. Vasc. Biol. 30, 39–45. doi: 10.1161/ATVBAHA.109.197442
Kimura, K., Nakamura, Y., Inaba, Y., Matsumoto, M., Kido, Y., Asahara, S., et al. (2013). Histidine augments the suppression of hepatic glucose production by central insulin action. Diabetes 62, 2266–2277. doi: 10.2337/db12-1701
Krishnappa, D., Sharma, S. K., Singh, A. D., Sinha, S., Ammini, A. C., Soneja, M. (2019). Impact of tuberculosis on glycaemic status: a neglected association. Indian J. Med. Res. 149, 384–388. doi: 10.4103/ijmr.IJMR_1927_17
Kubjane, M., Berkowitz, N., Goliath, R., Levitt, N. S., Wilkinson, R. J., Oni, T. (2020). Tuberculosis, human immunodeficiency virus, and the association with transient hyperglycemia in periurban south Africa. Clin. Infect. Dis. 71, 1080–1088. doi: 10.1093/cid/ciz928
Kumar, N. P., Babu, S. (2017). Influence of diabetes mellitus on immunity to human tuberculosis. Immunology 152, 13–24. doi: 10.1111/imm.12762
Kumar, N. P., Banurekha, V. V., Nair, D., Sridhar, R., Kornfeld, H., Nutman, T. B., et al. (2014). Coincident pre-diabetes is associated with dysregulated cytokine responses in pulmonary tuberculosis. PloS One 9, e112108. doi: 10.1371/journal.pone.0112108
Kumar, N. P., Fukutani, K. F., Shruthi, B. S., Alves, T., Silveira-Mattos, P. S., Rocha, M. S., et al. (2019). Persistent inflammation during anti-tuberculosis treatment with diabetes comorbidity. ELife 8, e46477. doi: 10.7554/eLife.46477
Kumar, N. P., Moideen, K., Dolla, C., Kumaran, P., Babu, S. (2017a). Prediabetes is associated with the modulation of antigen-specific Th1/Tc1 and Th17/Tc17 responses in latent Mycobacterium tuberculosis infection. PloS One 12, e0178000. doi: 10.1371/journal.pone.0178000
Kumar, N. P., Moideen, K., Sivakumar, S., Menon, P. A., Viswanathan, V., Kornfeld, H., et al. (2017b). Tuberculosis-diabetes co-morbidity is characterized by heightened systemic levels of circulating angiogenic factors. J. Infect. 74, 10–21. doi: 10.1016/j.jinf.2016.08.021
Kumpatla, S., Aravindalochanan, V., Rajan, R., Viswanathan, V., Kapur, A. (2013). Evaluation of performance of A1c and FPG tests for screening newly diagnosed diabetes defined by an OGTT among tuberculosis patients-a study from India. Diabetes Res. Clin. Pract. 102, 60–64. doi: 10.1016/j.diabres.2013.08.007
Laakso, M., Sarlund, H., Mykkänen, L. (1990). Insulin resistance is associated with lipid and lipoprotein abnormalities in subjects with varying degrees of glucose tolerance. Arteriosclerosis 10, 223–231. doi: 10.1161/01.atv.10.2.223
Lao, M., Li, C., Li, J., Chen, D., Ding, M., Gong, Y. (2020). Opportunistic invasive fungal disease in patients with type 2 diabetes mellitus from southern China: clinical features and associated factors. J. Diabetes. Investig. 11, 731–744. doi: 10.1111/jdi.13183
Lebovitz, H. E. (2001). Insulin resistance: definition and consequences. Exp. Clin. Endocrinol. Diabetes 109, S135–S148. doi: 10.1055/s-2001-18576
Lee, M. R., Huang, Y. P., Kuo, Y. T., Luo, C. H., Shih, Y. J., Shu, C. C., et al. (2017). Diabetes mellitus and latent tuberculosis infection: a systematic review and meta-analysis. Clin. Infect. Dis. 64, 719–727. doi: 10.1093/cid/ciw836
Li, P., Bandyopadhyay, G., Lagakos, W. S., Saswata, T., Osborn, O., Johnson, A. (2015). LTB4 promotes insulin resistance in obese mice by acting on macrophages, hepatocytes and myocytes. Nat. Med. 21, 239–247. doi: 10.1038/nm.3800
Li, C., Li, Q., Zhang, Y., Gong, Z., Ren, S., Li, P., et al. (2017). Characterization and function of Mycobacterium tuberculosis H37Rv lipase Rv1076 (LipU). Microbiol. Res. 196, 7–16. doi: 10.1016/j.micres.2016.12.005
Lijnen, H. R., Maquoi, E., Hansen, L. B., VanHoef, B., Frederix, L., Collen, D. (2002). Matrix metalloproteinase inhibition impairs adipose tissue development in mice. Arterioscler. Thromb. Vasc. Biol. 22, 374–379. doi: 10.1161/hq0302.104522
Lin, Y., Gong, J., Zhang, M., Xue, W., Barnes, P. F. (1998). Production of monocyte chemoattractant protein 1 in tuberculosis patients. Infect. Immun. 66, 2319–2322. doi: 10.1128/iai.66.5.2319-2322.1998
Lou, M., Luo, P., Tang, R., Peng, Y., Yu, S., Huang, W., et al. (2015). Relationship between neutrophil-lymphocyte ratio and insulin resistance in newly diagnosed type 2 diabetes mellitus patients. BMC Endocr. Disord. 15, 9. doi: 10.1186/s12902-015-0002-9
Lumeng, C. N., Saltiel, A. R. (2011). Inflammatory links between obesity and metabolic disease. J. Clin. Invest. 121, 2111–2117. doi: 10.1172/JCI57132
Luo, Y., Duan, H., Qian, Y., Feng, L., Wu, Z., Wang, F., et al. (2017). Macrophagic CD146 promotes foam cell formation and retention during atherosclerosis. Cell Res. 27, 352–372. doi: 10.1038/cr.2017.8
Lyons, C. L., Kennedy, E. B., Roche., H. R. (2016). Metabolic inflammation-differential modulation by dietary constituents. Nutrients 8, 247. doi: 10.3390/nu8050247
Magee, M. J., Khakharia, A., Gandhi, N. R., Day, C. L., Kornfeld, H., Rhee, M. K., et al. (2022). Increased risk of incident diabetes among individuals with latent tuberculosis infection. Diabetes Care 45, 880–887. doi: 10.2337/dc21-1687
Magee, M. J., Salindri, A. D., Kyaw, N. T. T., Auld, S. C., Haw, J. S., Umpierrez, G. E. (2018). Stress hyperglycemia in patients with tuberculosis disease: epidemiology and clinical implications. Curr. Diab. Rep. 18, 71. doi: 10.1007/s11892-018-1036-y
Mao, F., Chen, T., Zhao, Y., Zhang, C., Bai, B., Zhao, S., et al. (2011). Insulin resistance: a potential marker and risk factor for active tuberculosis? Med. Hypotheses 77, 66–68. doi: 10.1016/j.mehy.2011.03.025
Martinez, N., Cheng, C. Y., Ketheesan, N., Cullen, A., Tang, Y., Lum, J., et al. (2019). mTORC2/Akt activation in adipocytes is required for adipose tissue inflammation in tuberculosis. EBioMedicine 45, 314–327. doi: 10.1016/j.ebiom.2019.06.052
Martinez, N., Ketheesan, N., West, K., Vallerskog, T., Kornfeld, H. (2016). Impaired recognition of Mycobacterium tuberculosis by alveolar macrophages from diabetic mice. J. Infect. Dis. 214, 1629–1637. doi: 10.1093/infdis/jiw436
Martinez, N., Kornfeld, H. (2014). Diabetes and immunity to tuberculosis. Eur. J. Immunol. 44, 617–626. doi: 10.1002/eji.201344301
Masood, K. I., Irfan, M., Masood, Q., Yameen, M., Jamil, B., Ram, N., et al. (2022). Latent M. tuberculosis infection is associated with increased inflammatory cytokine and decreased suppressor of cytokine signalling (SOCS)-3 in the diabetic host. Scand. J. Immunol. 95, e13134. doi: 10.1111/sji.13134
Mayer-Barber, K. D., Sher, A. (2015). Cytokine and lipid mediator networks in tuberculosis. Immunol. Rev. 264, 264–275. doi: 10.1111/imr.12249
Means, T. K., Wang, S., Lien, E., Yoshimura, A., Golenbock, D. T., Fenton, M. J. (1999). Human toll-like receptors mediate cellular activation by Mycobacterium tuberculosis. J. Immunol. 163, 3920–3927. doi: 10.4049/jimmunol.163.7.3920
Meenakshi, P., Ramya, S., Lavanya, J., Vijayalakshmi, V., Sumanlatha, G. (2016). Effect of IFN-γ, IL-12 and IL-10 cytokine production and mRNA expression in tuberculosis patients with diabetes mellitus and their household contacts. Cytokine 81, 127–136. doi: 10.1016/j.cyto.2016.03.009
Menon, S., Rossi, R., Dusabimana, A., Zdraveska, N., Bhattacharyya, S., Francis, J. (2020). The epidemiology of tuberculosis-associated hyperglycemia in individuals newly screened for type 2 diabetes mellitus: systematic review and meta-analysis. BMC Infect. Dis. 20, 1–14. doi: 10.1186/s12879-020-05512-7
Mesquita, E. D., Gil-Santana, L., Ramalho, D., Tonomura, E., Silva, E. C., Oliveira, M., et al. (2016). Rede-TB study group. associations between systemic inflammation, mycobacterial loads in sputum and radiological improvement after treatment initiation in pulmonary TB patients from Brazil: a prospective cohort study. BMC Infect. Dis. 16, 368. doi: 10.1186/s12879-016-1736-3
Mishra, B. B., Lovewell, R. R., Olive, A. J., Zhang, G., Wang, W., Eugenin, E., et al. (2017). Nitric oxide prevents a pathogen-permissive granulocytic inflammation during tuberculosis. Nat. Microbiol. 2, 17072. doi: 10.1038/nmicrobiol.2017.72
Moideen, K., Kumar, N. P., Nair, D., Banurekha, V. V., Babu, S. (2018). Altered systemic adipokine levels in pulmonary tuberculosis and changes following treatment. Am. J. Trop. Med. Hyg. 99, 875–880. doi: 10.4269/ajtmh.18-0206
Molla, Y., Wubetu, M., Dessie, B. (2021). Anti-tuberculosis drug induced hepatotoxicity and associated factors among tuberculosis patients at selected hospitals, Ethiopia. Hepat. Med. 13, 1–8. doi: 10.2147/HMER.S290542
Moreira, J., Castro, R., Lamas, C., Ribeiro, S., Grinsztejn, B., Veloso, V. G. (2018). Hyperglycemia during tuberculosis treatment increases morbidity and mortality in a contemporary cohort of HIV-infected patients in Rio de Janeiro, Brazil. Int. J. Infect. Dis. 69, 11–19. doi: 10.1016/j.ijid.2017.12.014
Muefong, C. N., Sutherland, J. S. (2020). Neutrophils in tuberculosis-associated inflammation and lung pathology. Front. Immunol. 11. doi: 10.3389/fimmu.2020.00962
Mugusi, F., Swai, A. B., Alberti, K. G., McLarty, D. G. (1990). Increased prevalence of diabetes mellitus in patients with pulmonary tuberculosis in Tanzania. Tubercle 71, 271–276. doi: 10.1016/0041-3879(90)90040-f
Mukhopadhyay, S., Nair, S., Ghosh, S. (2012). Pathogenesis in tuberculosis: transcriptomic approaches to unraveling virulence mechanisms and finding new drug targets. FEMS Microbiol. Rev. 36, 463–485. doi: 10.1111/j.1574-6976.2011.00302.x
Mukhtar, F., Butt, Z. A. (2018). Risk of adverse treatment outcomes among new pulmonary TB patients co-infected with diabetes in Pakistan: a prospective cohort study. PloS One 13, e0207148. doi: 10.1371/journal.pone.0207148
Mupere, E., Malone, L., Zalwango, S., Okwera, A., Nsereko, M., Tisch, D. J., et al. (2014). Wasting among Uganda men with pulmonary tuberculosis is associated with linear regain in lean tissue mass during and after treatment in contrast to women with wasting who regain fat tissue mass: prospective cohort study. BMC Infect. Dis. 14, 24. doi: 10.1186/1471-2334-14-24
Nair, S., Ramaswamy, P. A., Ghosh, S., Joshi, D. C., Pathak, N., Siddiqui, I., et al. (2009). The PPE18 of Mycobacterium tuberculosis interacts with TLR2 and activates IL-10 induction in macrophage. J. Immunol. 183, 6269–6281. doi: 10.4049/jimmunol.0901367
Nathan, C., Shiloh, M. U. (2000). Reactive oxygen and nitrogen intermediates in the relationship between mammalian hosts and microbial pathogens. Proc. Natl. Acad. Sci. U.S. A. 97, 8841–8848. doi: 10.1073/pnas.97.16.8841
Navid, S., Sadegh-Ehdaei, B., Shabani, M., Hasani, M., Mirzaei, A., Ghazvini, K., et al. (2020). The case report of Mycobacterium arupense wound infection in diabetes mellitus patients; the first report and literature review. Access Microbiol. 2, acmi000106. doi: 10.1099/acmi.0.000106
Neyrolles, O., Hernández-Pando, R., Pietri-Rouxel, F., Fornès, P., Tailleux, L., Payán, J. A. B., et al. (2006). Is adipose tissue a place for Mycobacterium tuberculosis persistence? PloS One 1, e43. doi: 10.1371/journal.pone.0000043
Nezami, N., Ghorbanihaghjo, A., Rashtchizadeh, N., Argani, H., Tafrishinejad, A., Ghorashi, S., et al. (2011). Atherogenic changes of low-density lipoprotein susceptibility to oxidation, and antioxidant enzymes in pulmonary tuberculosis. Atherosclerosis. 217, 268–273. doi: 10.1016/j.atherosclerosis.2011.03.025
Niazi, A. K., Kalra, S. (2012). Diabetes and tuberculosis: a review of the role of optimal glycemic control. J. Diabetes Metab. Disord. 11, 28. doi: 10.1186/2251-6581-11-28
Nichols, G. P. (1957). Diabetes among young tuberculous patients; a review of the association of the two diseases. Am. Rev. Tuberc. 76, 1016–1030.
Niemi, M., Backman, J. T., Neuvonen, M., Neuvonen, P. J., Kivisto, K. T. (2001). Effects of rifampin on the pharmacokinetics and pharmacodynamics of glyburide and glipizide. Clin. Pharmacol. Ther. 69, 400–406. doi: 10.1067/mcp.2001.115822
Nijland, H. M. J., Ruslami, R., Stalenhoef, J. E., Nelwan, E. J., Alisjahbana, B., Nelwan, R. H. H., et al. (2006). Exposure to rifampicin is strongly reduced in patients with tuberculosis and type 2 diabetes. Clin. Infect. Dis. 43, 848–854. doi: 10.1086/507543
Oluboyo, P. O., Erasmus, R. T. (1990). The significance of glucose intolerance in pulmonary tuberculosis. Tuberculosis 71, 135–138. doi: 10.1016/0041-3879(90)90010-6
Omar, N., Wong, J., Thu, K., Alikhan, M. F., Chaw, L. (2021). Prevalence and associated factors of diabetes mellitus among tuberculosis patients in Brunei darussalam: a 6-year retrospective cohort study. Int. J. Infect. Dis. 105, 267–273. doi: 10.1016/j.ijid.2021.02.064
Ormazabal, V., Nair, S., Elfeky, O., Aguayo, C., Salomon, C., Zuñiga, F. A. (2018). Association between insulin resistance and the development of cardiovascular disease. Cardiovasc. Diabetol. 17, 122. doi: 10.1186/s12933-018-0762-4
Oswal, N., Lizardo, K., Dhanyalayam, D., Ayyappan, J. P., Thangavel, H., Heysell, S. K., et al. (2022). Host metabolic changes during Mycobacterium tuberculosis infection cause insulin resistance in adult mice. J. Clin. Med. 11, 1646. doi: 10.3390/jcm11061646
Pais, R., Zietek, T., Hauner, H., Daniel, H., Skurk, T. (2014). RANTES (CCL5) reduces glucose-dependent secretion of glucagon-like peptides 1 and 2 and impairs glucose-induced insulin secretion in mice. Am. J. Physiol. Gastrointest. Liver Physiol. 307, G330–G337. doi: 10.1152/ajpgi.00329.2013
Pajuelo, D., Gonzalez-Juarbe, N., Niederweis, M. (2020). NAD hydrolysis by the tuberculosis necrotizing toxin induces lethal oxidative stress in macrophages. Cell. Microbiol. 22, e13115. doi: 10.1111/cmi.13115
Pal, R., Bisht, M. K., Mukhopadhyay, S. (2022). Secretory proteins of Mycobacterium tuberculosis and their roles in modulation of host immune responses: focus on therapeutic targets. FEBS J. 289, 4146–4171. doi: 10.1111/febs.16369
Panee, J. (2012). Monocyte chemoattractant protein 1 (MCP-1) in obesity and diabetes. Cytokine 60, 1–12. doi: 10.1016/j.cyto.2012.06.018
Parveen, N., Varman, R., Nair, S., Das, G., Ghosh, S., Mukhopadhyay, S. (2013). Endocytosis of Mycobacterium tuberculosis heat shock protein 60 is required to induce interleukin-10 production in macrophages. J. Biol. Chem. 288, 24956–24971. doi: 10.1074/jbc.M113.461004
Peddinti, G., Cobb, J., Yengo, L., Froguel, P., Kravić, J., Balkau, B., et al. (2017). Early metabolic markers identify potential targets for the prevention of type 2 diabetes. Diabetologia 60, 1740–1750. doi: 10.1007/s00125-017-4325-0
Pérez-Torres, I., Gutiérrez-Alvarez, Y., Guarner-Lans, V., Díaz-Díaz, E., Manzano Pech, L., Caballero-Chacón, S. D. C. (2019). Intra-abdominal fat adipocyte hypertrophy through a progressive alteration of lipolysis and lipogenesis in metabolic syndrome rats. Nutrients 11, 1529. doi: 10.3390/nu11071529
Philips, L., Visser, J., Nel, D., Blaauw, R. (2017). The association between tuberculosis and the development of insulin resistance in adults with pulmonary tuberculosis in the western sub-district of the cape metropole region, south Africa: a combined cross-sectional, cohort study. BMC Infect. Dis. 17, 570. doi: 10.1186/s12879-017-2657-5
Piggott, D. A., Karakousis, P. C. (2011). Timing of antiretroviral therapy for HIV in the setting of TB treatment. J. Immunol. Res. 2011, 103917. doi: 10.1155/2011/103917
Prada-Medina, C. A., Fukutani, K. F., Kumar, N. P., Gil-Santana, L., Babu, S., Lichtenstein, F., et al. (2017). Systems immunology of diabetes-tuberculosis comorbidity reveals signatures of disease complications. Sci. Rep. 7, 1999. doi: 10.1038/s41598-017-01767-4
Qatanani, M., Szwergold, N. R., Greaves, D. R., Ahima, R. S., Lazar, M. A. (2009). Macrophage-derived human resistin exacerbates adipose tissue inflammation and insulin resistance in mice. J. Clin. Invest. 119, 531–519. doi: 10.1172/JCI37273
Quiding-Järbrink, M., Smith, D. A., Bancroft, G. J. (2001). Production of matrix metalloproteinases in response to mycobacterial infection. Infect. Immun. 69, 5661–5670. doi: 10.1128/IAI.69.9.5661-5670.2001
Rajaa, S., Krishnamoorthy, Y., Knudsen, S., Roy, G., Ellner, J., Horsburgh, C. R., et al. (2021). Prevalence and factors associated with diabetes mellitus among tuberculosis patients in south India–a cross-sectional analytical study. BMJ Open 11, e050542. doi: 10.1136/bmjopen-2021-050542
Rameshwaram, N. R., Singh, P., Ghosh, S., Mukhopadhyay, S. (2018). Lipid metabolism and intracellular bacterial virulence: key to next-generation therapeutics. Future Microbiol. 13, 1301–1328. doi: 10.2217/fmb-2018-0013
Ravimohan, S., Kornfeld, H., Weissman, D., Bisson, G. P. (2018). Tuberculosis and lung damage: from epidemiology to pathophysiology. Eur. Respir. Rev. 27, 170077. doi: 10.1183/16000617.0077-2017
Restrepo, B. I. (2016). Diabetes and tuberculosis. Microbiol. Spectr. 4, 10. doi: 10.1128/microbiolspec.TNMI7-0023-2016
Reyna, S. M., Ghosh, S., Tantiwong, P., Meka, C. S., Eagan, P., Jenkinson, C. P., et al. (2008). Elevated toll-like receptor 4 expression and signaling in muscle from insulin-resistant subjects. Diabetes 57, 2595–2602. doi: 10.2337/db08-0038
Rodríguez, Y., deArmas, Y., Capó, V., Wissmann, G., Goldani, L. Z., DeWaard, J. H. (2012). Sudden death related to tuberculous coronary arteritis. Int. J. Cardiol. 156, e28–e29. doi: 10.1016/j.ijcard.2011.08.002
Ruslami, R., Aarnoutse, R. E., Alisjahbana, B., van der Ven, A. J., vanCrevel, R. (2010). Implications of the global increase of diabetes for tuberculosis control and patient care. Trop. Med. Int. Health 15, 1289–1299. doi: 10.1111/j.1365-3156.2010.02625.x
Sama, L. F., Ali, I. M., Noubom, M., Nganou, D. O., Wam, E. C., et al. (2017). Liver dysfunction in TB-diabetic and TB non-diabetic patients admitted in TB referral hospital in western Cameroon. Biochem. Anal. Biochem. 6, 333. doi: 10.4172/2161-1009.1000333
Sami, W., Ansari, T., Butt, N. S., Hamid, M. R. A. (2017). Effect of diet on type 2 diabetes mellitus: a review. Int. J. Health Sci. 11, 65–71.
Saravanan, P., Dubey, V. K., Patra, S. (2012). Potential selective inhibitors against Rv0183 of Mycobacterium tuberculosis targeting host lipid metabolism. Chem. Biol. Drug Des. 79, 1056–1062. doi: 10.1111/j.1747-0285.2012.01373.x
Sartipy, P., Loskutoff, D. J. (2003). Monocyte chemoattractant protein 1 in obesity and insulin resistance. Proc. Natl. Acad. Sci. U. S. A. 100, 7265–7270. doi: 10.1073/pnas.1133870100
Sathkumara, H. D., Muruganandah, V., Cooper, M. M., Field, M. A., Alim, M. A., Brosch, R., et al. (2020). Mucosal delivery of ESX-1-expressing BCG strains provides superior immunity against tuberculosis in murine type 2 diabetes. Proc. Natl. Acad. Sci. U S A. 17, 20848–20859. doi: 10.1073/pnas.2003235117
Schofield, J. D., Liu, Y., Rao-Balakrishna, P., Malik, R. A., Soran, H. (2016). Diabetes dyslipidemia. Diabetes Ther. 7, 203–219. doi: 10.1007/s13300-016-0167-x
Schué, M., Maurin, D., Dhouib, R., N’Goma, J.-C. B., Delorme, V., Lambeau, G., et al. (2010). Two cutinase-like proteins secreted by Mycobacterium tuberculosis show very different lipolytic activities reflecting their physiological function. FASEB J. 24, 1893–1903. doi: 10.1096/fj.09-144766
Sears, B., Perry, M. (2015). The role of fatty acids in insulin resistance. Lipids Health Dis. 14, 121. doi: 10.1186/s12944-015-0123-1
Selby, N. M., Taal, M. W. (2020). An updated overview of diabetic nephropathy: diagnosis, prognosis, treatment goals and latest guidelines. Diabetes Obes. Metab. 22, 3–15. doi: 10.1111/dom.14007
Sewter, C., Berger, D., Considine, R. V., Medina, G., Rochford, J., Ciaraldi, T., et al. (2002). Human obesity and type 2 diabetes are associated with alterations in SREBP1 isoform expression that are reproduced ex vivo by tumor necrosis factor-alpha. Diabetes. 51, 1035–1041. doi: 10.2337/diabetes.51.4.1035
Shen, G., Singh, K., Chandra, D., Serveau-Avesque, C., Maurin, D., Canaan, S., et al. (2012). LipC (Rv0220) is an immunogenic cell surface esterase of Mycobacterium tuberculosis. Infect. Immun. 80, 243–253. doi: 10.1128/IAI.05541-11
Shi, L., Jiang, Q., Bushkin, Y., Subbian, S., Tyagi, S. (2019). Biphasic dynamics of macrophage immunometabolism during Mycobacterium tuberculosis infection. MBio 10, e02550–e02518. doi: 10.1128/mBio.02550-18
Shi, L., Salamon, H., Eugenin, E. A., Pine, R., Cooper, A., Gennaro, M. L. (2015). Infection with Mycobacterium tuberculosis induces the warburg effect in mouse lungs. Sci. Rep. 5, 1–13. doi: 10.1038/srep18176
Shvets, O., Shevchenko, O., Piskur, Z., Stepanenko, H., Pohorielova, O. (2021). Pro-atherogenic lipid profile in pulmonary tuberculosis patients with concurrent insulin resistance. Inter. Collegas. 8, 111–114. doi: 10.35339/ic.8.2.111-114
Shvets, O. M., Shevchenko, O. S., Stepanenko, H. L. (2019). Insulin resistance in drug-susceptible pulmonary tuberculosis patients during the first month of antitubercular treatment. Int. Acad. J. Web Sch. 1, 13–17. doi: 10.31435/rsglobal_wos/31082019/6650
Silswal, N., Singh, A. K., Aruna, B., Mukhopadhyay, S., Ghosh, S., Ehtesham, N. Z. (2005). Human resistin stimulates the pro-inflammatory cytokines TNF-alpha and IL-12 in macrophages by NF-kappaB-dependent pathway. Biochem. Biophys. Res. Commun. 334, 1092–1101. doi: 10.1016/j.bbrc.2005.06.202
Singh, K. H., Jha, B., Dwivedy, A., Choudhary, E., AG, N., Ashraf, A., et al. (2017). Characterization of a secretory hydrolase from Mycobacterium tuberculosis sheds critical insight into host lipid utilization by M. tuberculosis. J. Biol. Chem. 292, 11326–11335. doi: 10.1074/jbc.M117.794297
Singh, V., Kaur, C., Chaudhary, V. K., Rao, K. V. S., Chatterjee, S. (2015). M. tuberculosis secretory protein ESAT-6 induces metabolic flux perturbations to drive foamy macrophage differentiation. Sci. Rep. 5, 1–12. doi: 10.1038/srep12906
Sinha, R., Ngo, M. D., Bartlett, S., Bielefeldt-Ohmann, H., Keshvari, S., Hasnain, S. Z., et al. (2021). Pre-diabetes increases tuberculosis disease severity, while high body fat without impaired glucose tolerance is protective. Front. Cell. Infect. Microbiol. 11. doi: 10.3389/fcimb.2021.691823
Solinas, G., Vilcu, C., Neels, J. G., Bandyopadhyay, G. K., Luo, J. L., Naugler, W., et al. (2007). JNK1 in hematopoietically derived cells contributes to diet-induced inflammation and insulin resistance without affecting obesity. Cell Metab. 6, 386–397. doi: 10.1016/j.cmet.2007.09.011
Song, M. J., Kim, K. H., Yoon, J. M., Kim, J. B. (2006). Activation of toll-like receptor 4 is associated with insulin resistance in adipocytes. Biochem. Biophys. Res. Commun. 346, 739–745. doi: 10.1016/j.bbrc.2006.05.170
Spranger, J., Kroke, A., Möhlig, M., Hoffmann, K., Bergmann, M. M., Ristow, M., et al. (2003). Inflammatory cytokines and the risk to develop type 2 diabetes: results of the prospective population-based European prospective investigation into cancer and nutrition (EPIC)-potsdam study. Diabetes 52, 812–817. doi: 10.2337/diabetes.52.3.812
Ssekamatte, P., Nakibuule, M., Nabatanzi, R., Egesa, M., Musubika, C., Bbuye, M., et al. (2021). Type 2 diabetes mellitus and latent tuberculosis infection moderately influence innate lymphoid cell immune responses in Uganda. Front. Immunol. 12. doi: 10.3389/fimmu.2021.716819
Stalenhoef, J. E., Alisjahbana, B., Nelwan, E. J., vanderVen-Jongekrijg, J., Ottenhoff, T. H., vanderMeer, J. W., et al. (2008). The role of interferon-gamma in the increased tuberculosis risk in type 2 diabetes mellitus. Eur. J. Clin. Microbiol. Infect. Dis. 27, 97–103. doi: 10.1007/s10096-007-0395-0
Sun, K., Kusminski, C. M., Scherer, P. E. (2011). Adipose tissue remodeling and obesity. J. Clin. Invest. 121, 2094–2101. doi: 10.1172/JCI45887
Suzuki, M., Shinozaki, K., Kanazawa, A., Hara, Y., Hattori, Y., Tsushima, M., et al. (1996). Insulin resistance as an independent risk factor for carotid wall thickening. Hypertension 28, 593–598. doi: 10.1161/01.HYP.28.4.593
Takasu, N., Yamada, T., Miura, H., Sakamoto, S., Korenaga, M., Nakajima, K., et al. (1982). Rifampicin-induced early phase hyperglycemia in humans. Am. Rev. Respir. Dis. 125, 23–27. doi: 10.1164/arrd.1982.125.1.23
Tan, S. Y., Wong, J. L. M., Sim, Y. J., Wong, S. S., Elhassan, S. A. M., Tan, S. H., et al. (2019). Type 1 and 2 diabetes mellitus: a review on current treatment approach and gene therapy as potential intervention. Diabetes Metab. Syndr. 13, 364–372. doi: 10.1016/j.dsx.2018.10.008
Tater, D., Tepaut, B., Bercovici, J. P., Youinou, P. (1987). Polymorphonuclear cell derangements in type I diabetes. Horm. Metab. Res. 19, 642–647. doi: 10.1055/s-2007-1011899
Tegegne, B. S., Mengesha, M. M., Teferra, A. A., Awoke, M. A., Habtewold, T. D. (2018). Association between diabetes mellitus and multi-drug-resistant tuberculosis: evidence from a systematic review and meta-analysis. Syst. Rev. 7, 161. doi: 10.1186/s13643-018-0828-0
Tesch, G. H. (2008). MCP-1/CCL2: a new diagnostic marker and therapeutic target for progressive renal injury in diabetic nephropathy. Am. J. Physiol. Renal. Physiol. 294, 697–701. doi: 10.1152/ajprenal.00016.2008
Tsalamandris, S., Antonopoulos, A. S., Oikonomou, E., Papamikroulis, G. A., Vogiatzi, G., Papaioannou, S., et al. (2019). The role of inflammation in diabetes: current concepts and future perspectives. Eur. Cardiol. 14, 50–59. doi: 10.15420/ecr.2018.33.1
Udgata, A., Dolasia, K., Ghosh, S., Mukhopadhyay, S. (2019). Dribbling through the host defense: targeting the TLRs by pathogens. Crit. Rev. Microbiol. 45, 354–368. doi: 10.1080/1040841X.2019.1608904
Vallerskog, T., Martens, G. W., Kornfeld, H. (2010). Diabetic mice display a delayed adaptive immune response to. Mycobacterium tuberculosis. J. Immunol. 184, 6275–6282. doi: 10.4049/jimmunol.1000304
vanCrevel, R., Critchley, J. A. (2021). The interaction of diabetes and tuberculosis: translating research to policy and practice. Trop. Med. Infect. Dis. 6, 8. doi: 10.3390/tropicalmed6010008
vanCrevel, R., Karyadi, E., Netea, M. G., Verhoef, H., Nelwan, R. H., West, C. E., et al. (2002). Decreased plasma leptin concentrations in tuberculosis patients are associated with wasting and inflammation. J. Clin. Endocrinol. Metab. 87, 758–763. doi: 10.1210/jcem.87.2.8228
Viswanathan, V., Kumpatla, S., Aravindalochanan, V., Rajan, R., Chinnasamy, C., Srinivasan, R., et al. (2012). Prevalence of diabetes and pre-diabetes and associated risk factors among tuberculosis patients in India. PloS One 7, 1–9. doi: 10.1371/journal.pone.0041367
Viswanathan, V., Vigneswari, A., Selvan, K., Satyavani, K., Rajeswari, R., Kapur, A. (2014). Effect of diabetes on treatment outcome of smear-positive pulmonary tuberculosis–a report from south India. J. Diabetes Complicat. 28, 162–165. doi: 10.1016/j.jdiacomp.2013.12.003
Vrieling, F., Alisjahbana, B., Sahiratmadja, E., Crevel, C. V., Amy, C. H., Hankemeier, H., et al. (2019). Plasma metabolomics in tuberculosis patients with and without concurrent type 2 diabetes at diagnosis and during antibiotic treatment. Sci. Rep. 9, 18669. doi: 10.1038/s41598-019-54983-5
Vrieling, F., Ronacher, K., Kleynhans, L., van den Akker, E., Walzl, G., Ottenhoff, T. H. M., et al. (2018). Patients with concurrent tuberculosis and diabetes have a pro-atherogenic plasma lipid profile. EBioMedicine 32, 192–200. doi: 10.1016/j.ebiom.2018.05.011
Wang, X., Hu, Z., Hu, J., Du, J., Mitch, W. E. (2006). Insulin resistance accelerates muscle protein degradation: activation of the ubiquitin-proteasome pathway by defects in muscle cell signaling. Endocrinology 147, 4160–4168. doi: 10.1210/en.2006-0251
Wang, L., Hu, J., Zhou, H. (2020). Macrophage and adipocyte mitochondrial dysfunction in obesity-induced metabolic diseases. World J. Men’s Health 38, 606–614. doi: 10.5534/wjmh.200163
Wang, L., Liu, Z., Wang, J., Liu, H., Wu, J., Tang, T., et al. (2019). Oxidization of TGFβ-activated kinase by MPT53 is required for immunity to. Mycobacterium tuberculosis. Nat. Microbiol. 4, 1378–1388. doi: 10.1038/s41564-019-0436-3
Wang, Q., Ma, A., Han, X., Zhao, S., Cai, J., Kok, F. J., et al. (2017). Hyperglycemia is associated with increased risk of patient delay in pulmonary tuberculosis in rural areas. J. Diabetes 9, 648–655. doi: 10.1111/1753-0407.12459
Waterhouse, M., Wilson, C., White, V. L., Chowdhury, T. A. (2005). Resolution of insulin-requiring diabetes after cessation of chemotherapy for tuberculosis. J. R. Soc Med. 98, 270–271. doi: 10.1177/014107680509800607
Wei, R., Li, P., Xue, Y., Liu, Y., Gong, W., Zhao, W. (2022). Impact of diabetes mellitus on the immunity of tuberculosis patients: a retrospective, cross-sectional study. Risk Manag Healthc Policy. 15, 611–627. doi: 10.2147/RMHP.S354377
Weiner, J., Maertzdorf, J., Sutherland, J. S., Fergal, J. D., Thompson, E., Suliman, S., et al. (2018). Metabolite changes in blood predict the onset of tuberculosis. Nat. Commun. 9, 5208. doi: 10.1038/s41467-018-07635-7
Weiner, J., Parida, S. K., Maertzdorf, J., Black, G. F., Repsilber, D., Telaar, A., et al. (2012). Biomarkers of inflammation, immunosuppression and stress are revealed by metabolomic profiling of tuberculosis patients. PloS One 7, e40221. doi: 10.1371/journal.pone.0040221
Williams, V., Onwuchekwa, C., Vos, A. G., Grobbee, D. E., Otwombe, K., Klipstein-Grobusch, K. (2022). Tuberculosis treatment and resulting abnormal blood glucose: a scoping review of studies from 1981 - 2021. Glob. Health Action. 15, 2114146. doi: 10.1080/16549716.2022.2114146
Workneh, M. H., Bjune, G. A., Yimer, S. A. (2016). Diabetes mellitus is associated with increased mortality during tuberculosis treatment: a prospective cohort study among tuberculosis patients in south-Eastern amahra region, Ethiopia. Infect. Dis. Poverty 5, 22. doi: 10.1186/s40249-016-0115-z
Workneh, M. H., Bjune, G. A., Yimer, S. A. (2017). Prevalence and associated factors of tuberculosis and diabetes mellitus comorbidity: a systematic review. PloS One 12, e0175925. doi: 10.1371/journal.pone.0175925
World Health Organization (2022). Global tuberculosis report 2022 (Geneva: World Health Organization). Available at: https://apps.who.int/iris/handle/10665/363752.
Xin, Y., Wang, Y., Chi, J., Zhu, X., Zhao, H., Zhao, S., et al. (2019). Elevated free fatty acid level is associated with insulin-resistant state in nondiabetic Chinese people. Diabetes Metab. Syndr. Obes. 12, 139–147. doi: 10.2147/DMSO.S186505
Xu, G., Jia, H., Li, Y., Liu, X., Li, M., Wang, Y. (2010). Hemolytic phospholipase Rv0183 of Mycobacterium tuberculosis induces inflammatory response and apoptosis in alveolar macrophage RAW264.7 cells. Can. J. Microbiol. 56, 916–924. doi: 10.1139/W10-079
Yamashiro, S., Kawakami, K., Uezu, K., Kinjo, T., Miyagi, K., Nakamura, K., et al. (2005). Lower expression of Th1-related cytokines and inducible nitric oxide synthase in mice with streptozotocin-induced diabetes mellitus infected with Mycobacterium tuberculosis. Clin. Exp. Immunol. 139, 57–64. doi: 10.1111/j.1365-2249.2005.02677.x
Yorke, E., Atiase, Y., Akpalu, J., Sarfo-Kantanka, O., Boima, V., Dey, I. D. (2017). The bi-directional relationship between tuberculosis and diabetes. Tuberc. Res. Treat. 2017, 1702578. doi: 10.1155/2017/1702578
Youn, B. S., Yu, K. Y., Park, H. J., Lee, N. S., Min, S. S., Youn, M. Y., et al. (2004). Plasma resistin concentrations measured by enzyme-linked immunosorbent assay using a newly developed monoclonal antibody are elevated in individuals with type 2 diabetes mellitus. J Clin Endocrinol Metab. 89, 150–156. doi: 10.1210/jc.2003-031121
Yurt, S., Erman, H., Korkmaz, G. G., Kosar, A. F., Uysal, P., Gelisgen, R., et al. (2013). The role of feed regulating peptides on weight loss in patients with pulmonary tuberculosis. Clin. Biochem. 46, 40–44. doi: 10.1016/j.clinbiochem.2012.09.008
Zhang, H. H., Halbleib, M., Ahmad, F., Manganiello, V. C., Greenberg, A. S. (2002). Tumor necrosis factor-α stimulates lipolysis in differentiated human adipocytes through activation of extracellular signal-related kinase and elevation of intracellular cAMP. Diabetes 51, 2929–2935. doi: 10.2337/diabetes.51.10.2929
Keywords: Mycobacterium tuberculosis, insulin resistance, diabetes, inflammation, therapeutic strategies
Citation: Bisht MK, Dahiya P, Ghosh S and Mukhopadhyay S (2023) The cause–effect relation of tuberculosis on incidence of diabetes mellitus. Front. Cell. Infect. Microbiol. 13:1134036. doi: 10.3389/fcimb.2023.1134036
Received: 29 December 2022; Accepted: 25 May 2023;
Published: 26 June 2023.
Edited by:
Khushboo Borah Slater, University of Surrey, United KingdomReviewed by:
Subhadip Choudhuri, University of Texas Medical Branch at Galveston, United StatesSrijon Kaushik Banerjee, University of Pittsburgh, United States
Copyright © 2023 Bisht, Dahiya, Ghosh and Mukhopadhyay. This is an open-access article distributed under the terms of the Creative Commons Attribution License (CC BY). The use, distribution or reproduction in other forums is permitted, provided the original author(s) and the copyright owner(s) are credited and that the original publication in this journal is cited, in accordance with accepted academic practice. No use, distribution or reproduction is permitted which does not comply with these terms.
*Correspondence: Sangita Mukhopadhyay, sangita@cdfd.org.in