MicroRNAs in the Regulation of Solute Carrier Proteins Behind Xenobiotic and Nutrient Transport in Cells
- Department of Biochemistry and Molecular Medicine, School of Medicine, University of California, Davis, Sacramento, CA, United States
Altered metabolism, such as aerobic glycolysis or the Warburg effect, has been recognized as characteristics of tumor cells for almost a century. Since then, there is accumulating evidence to demonstrate the metabolic reprogramming of tumor cells, addiction to excessive uptake and metabolism of key nutrients, to support rapid proliferation and invasion under tumor microenvironment. The solute carrier (SLC) superfamily transporters are responsible for influx or efflux of a wide variety of xenobiotic and metabolites that are needed for the cells to function, as well as some medications. To meet the increased demand for nutrients and energy, SLC transporters are frequently dysregulated in cancer cells. The SLCs responsible for the transport of key nutrients for cancer metabolism and energetics, such as glucose and amino acids, are of particular interest for their roles in tumor progression and metastasis. Meanwhile, rewired metabolism is accompanied by the dysregulation of microRNAs (miRNAs or miRs) that are small, noncoding RNAs governing posttranscriptional gene regulation. Studies have shown that many miRNAs directly regulate the expression of specific SLC transporters in normal or diseased cells. Changes of SLC transporter expression and function can subsequently alter the uptake of nutrients or therapeutics. Given the important role for miRNAs in regulating disease progression, there is growing interest in developing miRNA-based therapies, beyond serving as potential diagnostic or prognostic biomarkers. In this article, we discuss how miRNAs regulate the expression of SLC transporters and highlight potential influence on the supply of essential nutrients for cell metabolism and drug exposure toward desired efficacy.
Introduction
The first microRNA (miRNA), namely lin-4, was originally discovered in Caenorhabditis elegans in 1993 (Lee et al., 1993; Wightman et al., 1993). It was found that lin-4 suppressed the translation of lin-14 through complementary base pairing, and lin-4 function is crucial for larval development (Lee et al., 1993; Wightman et al., 1993). Since then, functional miRNAs are revealed as a superfamily of noncoding RNAs (ncRNAs) in almost all species, including humans (Pasquinelli et al., 2000). About 18–25 nucleotides in length, genome-derived miRNAs generally act on the 3′-untranslated region (3′UTR) of target mRNAs to control posttranscriptional gene expression (Ambros, 2004; Bartel, 2004; Friedman et al., 2009). As a result, miRNAs are crucial regulators of essentially all cellular processes, and dysregulation of some miRNAs may be associated with specific diseases. Indeed, many miRNAs are involved in the regulation of cancer cell properties, including cell cycle, proliferation, apoptosis, metabolism, senescence, stemness, and immunity as well as xenobiotic drug metabolism and disposition (Yu and Pan, 2012; Yu et al., 2016), whereas some miRNAs are dysregulated in cancer cells (Anfossi et al., 2018; Dragomir et al., 2021). With an improved understanding of miRNA functions and cancer biology, new miRNA-based therapeutic strategies are emerging for the treatment of various types of cancer diseases (Rupaimoole and Slack, 2017; Yu A.-M. et al., 2020; Kara et al., 2022).
The dysregulated uptake and metabolism of key nutrients, such as glucose and amino acids (AAs), has been identified as a hallmark of cancer (Hanahan and Weinberg, 2011; Pavlova and Thompson, 2016). Among them, the influx and efflux of nutrients are mediated by solute carrier (SLC) transporters, and some SLCs have been characterized as either tumor suppressors or promoters with roles in many cellular processes (Rashid et al., 2021). SLCs are facilitative transporters or secondary active transporters but do not use ATP for energy as ATP-binding cassette (ABC) transporters do (Pizzagalli et al., 2021). In addition to essential nutrients, ions, and endobiotic metabolites (Zhang et al., 2019), some SLCs are also involved in the transport of therapeutics and their metabolites (Zhou et al., 2017). Meanwhile, some miRNAs are also revealed to modulate cancer cell metabolism (Pedroza-Torres et al., 2019) through the direct targeting of specific nutrient metabolic enzymes, in addition to a variety of SLC transporters. Interestingly, even though SLC proteins are the largest group of transporters, with over 450 members, SLCs are understudied considering their critical roles in the transport of essential nutrients and metabolites as well as medications and toxins pivotal to physiology, disease etiology, and pharmacotherapy (César-Razquin et al., 2015).
In this article, we will provide an overview of miRNA-controlled regulation of SLC family transporters involved in the transport and homeostasis of nutrients (e.g., carbohydrates and AAs) that are critical for cell survival with a focus on those related to cancer cell metabolism, as well as SLC drug transporters that are important for clinical therapy. How miRNA dysregulation can reprogram cancer cell metabolism will be highlighted. Furthermore, we will discuss the possibility and strategies for applying miRNA cancer biology, including miRNA–SLC interactions, to the development of new anticancer therapies.
MicroRNA Biogenesis and Functions
The canonical miRNA biogenesis pathway begins with the transcription of the primary miRNA (pri-miRNA) by RNA polymerase II within the nucleus (Figure 1). DROSHA and the cofactor DiGeorge Syndrome Critical Region 8 (DGCR8) form microprocessor to cut the pri-miRNA into a shorter precursor miRNA (pre-miRNA) (Denli et al., 2004). Pre-miRNA is exported into the cytoplasm through binding with exportin 5 and Ran-GTP. The pre-miRNA is then cleaved by DICER to the miRNA duplex, in which the guide strand is loaded into the RNA-induced silencing complex (RISC) consisting of Argonaute (AGO) family proteins, and the passenger strand is degraded (Schwarz et al., 2003) (Figure 1). There are additional non-canonical miRNA biogenesis pathways, which can be described as DROSHA/DGCR8-independent and/or DICER-independent (O'Brien et al., 2018). The active miRNA within RISC thus acts on the 3′UTR of its target mRNA to inhibit translation or increase the cleavage and degradation of the mRNA to achieve posttranscriptional gene regulation (Figure 1). Interestingly, a single miRNA may target many transcripts (Brennecke et al., 2005) so that miRNA dysregulation can have widespread effects in diseased cells. Indeed, miRNAs are involved in the regulation of almost all cellular processes including cancer cell tumorigenesis, progression, and metastasis, in which miRNAs may act as either tumor suppressors or oncomiRs, due to the ability to regulate most protein-coding genes (Dragomir et al., 2021).
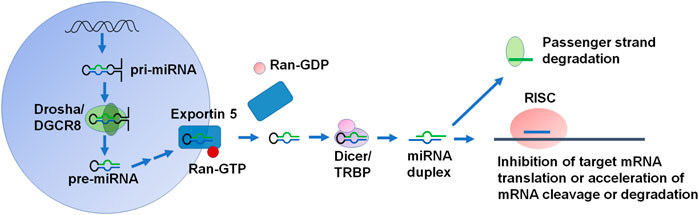
FIGURE 1. The canonical biogenesis and function of miRNAs in cells. Canonical miRNA biogenesis starts from the production of primary miRNA (pri-miRNA) transcript from DNA primarily by RNA polymerase II, which is processed subsequently by DROSHA to precursor miRNA (pre-miRNA) within the nucleus. Pre-miRNA is exported into the cytoplasm by Exportin 5. In the cytoplasm, the pre-miRNA detaches from Exportin 5/Ran-GDP and then cleaved by DICER to offer miRNA duplex. After the miRNA duplex is unwound, the passenger strand is degraded while the guide strand or mature miRNA is preferably loaded into the RISC. Functional miRNA within the RISC binds to the complementary MRE site in the 3′UTR of target transcript to inhibit mRNA translation or accelerate its cleavage or degradation to achieve posttranscriptional gene regulation.
Multiple factors influence miRNA expression or function. Most miRNA coding genes are in fragile sites or cancer-associated regions (Calin et al., 2004). MiRNA may be dysregulated by multiple mechanisms including gene deletions, amplifications, and mutations as well as alterations of relevant transcription factors, binding proteins, epigenetics, and posttranscriptional modifications (Dragomir et al., 2021). Indeed, many miRNAs have been reported as downregulated in cancer cells (Lu et al., 2005). One relating factor is the changes in DROSHA or DICER levels in specific cancers (Hata and Kashima, 2016; Ali Syeda et al., 2020). Furthermore, circular RNAs (circRNAs) have been widely reported as miRNA sponges that may prevent miRNA binding to their mRNA targets (Yarmishyn et al., 2022). The dysregulation of miRNA has been proven to influence all hallmarks of cancer (Peng and Croce, 2016; Van Roosbroeck and Calin, 2017).
Cancer Cell Metabolism and Roles of SLC Transporters
Nutrients including carbohydrates (e.g., glucose), amino acids (e.g., glutamine), and vitamins (e.g., folate) are crucial for cell survival. The supply and metabolism of these nutrients (Figure 2) provide building blocks for other essential molecules (e.g., ATP and nucleotides) as well as providing reducing power from NADH, NADPH, and FADH2, which are dependent upon many respective SLC transporters and metabolic enzymes. Diseased cells such as carcinoma cells are faced with both a nutrient-poor environment and increased demands for growth and proliferation, which necessitates changes in gene regulation to reprogram nutrient metabolism to maintain necessary energetic and metabolite supplies (Pavlova and Thompson, 2016; Wei et al., 2020; Papalazarou and Maddocks, 2021).
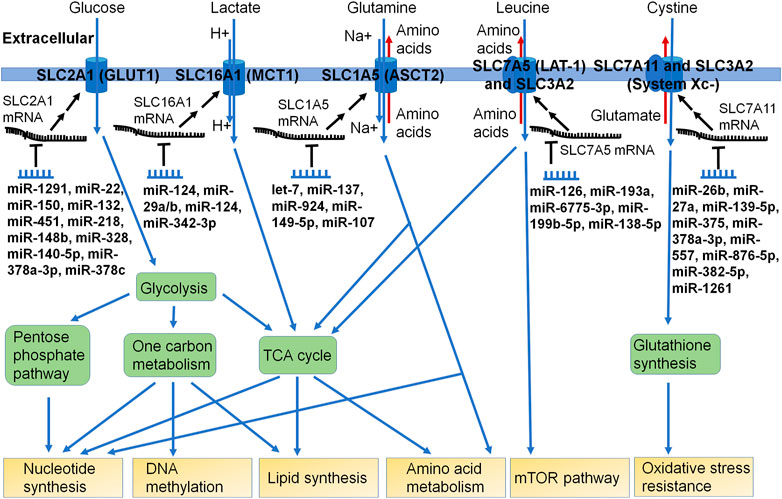
FIGURE 2. Many miRNAs have been shown to control the expression of specific SLC transporters critical for nutrient uptake or efflux in cells. The SLC transporters fuel multiple metabolic pathways, such as aminolyses, glycolysis, pentose phosphate pathway, one carbon metabolism, and TCA cycle. Some miRNAs have been identified to directly modulate the posttranscriptional gene expression of SLC transporters, which can impact the supply and homeostasis of nutrients or metabolic building blocks crucial for cell survival and growth. As certain SLC transporters are involved in the influx or efflux of xenobiotic drugs or toxins, the significance of the miRNA-controlled regulation of such SLCs in xenobiotic disposition and pharmacotherapy remains elusive.
The SLC superfamily encompasses over 450 proteins in humans, where proteins with at least 20% sequence similarity are grouped into a family (Hediger et al., 2013; Schumann et al., 2020). A list of SLCs and their characteristics, such as transport type, substrates, and localization, can be accessed through the Bioparadigms SLC Tables website (https://www.bioparadigms.org/slc/intro.htm), which has been validated by the HUGO Gene Nomenclature Committee (HGNC) (Hediger et al., 2013). Interestingly, some SLCs in different families lack sequence similarity but still have similar substrates. For instance, the SLC25 family members, which are present in both prokaryotes and eukaryotes, do not have significant sequence similarity to the SLC1A family; however, SLC25A22 and SLC1A1 are both glutamate transporters (Schlessinger et al., 2010). This similarity in function despite sequence differences is likely due to convergent evolution (Schlessinger et al., 2010). From evolutionary analysis based on the sequence, it has been found that 59% of human SLCs are also present in prokaryotes and the common ancestor of Eukaryotes, Eubacteria, and Archaea had SLCs (Hoglund et al., 2011).
SLCs are either passive facilitative transporters, which allows a substrate to move down its gradient, or secondary active transporters, where one substrate travels down its gradient to fuel the transport of another substrate against its gradient. Many transcription factors are known to regulate SLC levels in cancer; for instance, MYC and HIF1α are predicted to target SLC38A1 (Bröer et al., 2016; Panda et al., 2020). MiRNAs have important roles in posttranscriptional regulation, whose interactions with SLCs will be reviewed in further sections. Some common posttranslational modifications include N-glycosylation, palmitoylation, acetylation for control over mitochondrial SLC activity, phosphorylation to control transport activity, SUMOylation, and ubiquitination for degradation (Czuba et al., 2018). SLCs are predicted to have between 1 and 16 transmembrane domains based on hydropathy plots, and most known structures have transmembrane pseudosymmetry. Nevertheless, there are just a limited number of high-resolution structures due to challenges in purifying and analyzing transmembrane proteins (Bai et al., 2017; Pizzagalli et al., 2021). Over 80 SLCs have been associated with monogenic disorders, exemplifying their crucial role in human health and metabolism (Lin et al., 2015).
As revealed by Warburg (Warburg, 1956), there is an increase in glucose use and subsequent lactate production, known as aerobic glycolysis, in cancer cells even in the presence of oxygen. Many changes are linked to the Warburg effect, including an increase in the SLC2A family of glucose transporters, glycolytic enzymes, lactate dehydrogenase, SLC16A family of lactate transporters, and flow into the pentose-phosphate pathway (PPP) (Kozal et al., 2021). For example, elevated SLC2A1 (GLUT1) and SLC2A3 (GLUT3) have been associated with increased cancer metabolism (Ancey et al., 2018). The SLC16A family lactate transporters have also been reported to be dysregulated, in part to maintain intracellular pH for continued growth with increased lactate production (Felmlee et al., 2020).
The PPP consists of the non-oxidative and the oxidative branches. The non-oxidative branch produces ribose 5-phosphate, while the oxidative branch is necessary for NADPH and ribulose 5-phosphate production. Ribose 5-phosphate can then be used for nucleotide synthesis. Cancer cells with high SLC7A11 import more cystines, which is converted into cysteine with the use of NADPH for glutathione synthesis. This results in PPP dependency to maintain NADPH levels for redox homeostasis (Liu et al., 2020). Glucose starvation or SLC2A inhibition with KL-11743 results in cell death due to insufficient NADPH (Liu et al., 2020).
The metabolite 3-phosphoglyceric acid from glycolysis may be converted into serine for use in one-carbon metabolism (Figure 2). In HEK293T cells, half of the serine was shown to be synthesized from glucose (Locasale et al., 2011). Other possible sources of carbon for one-carbon metabolism include glycine and threonine (Locasale, 2013). One-carbon metabolism is made of the folate and methionine cycle. The folate cycle produces 10-formyl-THF, which can be used in purine synthesis. The methionine cycle produces S-adenosylmethionine, which can be used by methyltransferases. By altering the use of glucose in metabolic pathways, cancer cells can increase the production of building blocks that are not in as high of demand for cellular senescence.
The Warburg effect reduces glucose-derived pyruvate levels, so the TCA cycle also relies on glutaminolysis to supply alpha-ketoglutarate. In the TCA cycle, citrate can be converted into acetyl-CoA, which will serve as precursors for fatty acid synthesis (Figure 2). Malate can be exported into the cytoplasm to produce pyruvate and NADPH. Oxaloacetate can be converted into aspartate for nucleotide synthesis. Two important roles of glutamine are conversion into lactate for NADPH production necessary for fatty acid synthesis and TCA cycle anaplerosis (Deberardinis et al., 2007). SLC1A5 (ASCT2) is a highly studied transporter of glutamine (Bröer, 2020). After entry into the cell, glutamine can be converted into glutamate by glutaminase. Glutamate may then be converted into alpha-ketoglutarate by glutamate dehydrogenase or processed by transaminases, which will lead to the production of nonessential amino acids (Figure 2) (Yang et al., 2017).
SLC7A5 (LAT1) with SLC3A2 is another crucial amino acid transporter of essential amino acids, including leucine. Lysosomal-associated transmembrane protein 4b (LAPTM4b) has been shown to bind to SLC7A5/SLC3A2 to bring it to the lysosomal membrane, which is crucial for leucine to enter the lysosome to activate the mammalian target of rapamycin 1 (mTORC1) (Milkereit et al., 2015). Activation of mTORC1 in cancer cells results in tumor growth, survival, and metastasis by stimulating synthesis pathways such as for nucleotide, lipid, and protein (Hua et al., 2019). To maintain these metabolic processes, sufficient nutrients must be transported into the cells in which SLC transporters are largely involved (Figure 2).
Carbohydrate Transporters and MicroRNA Regulation
The SLC2 (glucose transporter or GLUT) and SLC5 family proteins are critical for the transport of carbohydrates across the cell membrane (Papalazarou and Maddocks, 2021). For instance, a crucial role documented for transporters SLC2A1-4 or GLUT1-4 is to facilitate the passive transport of glucose. After import into the cell, glucose is phosphorylated by hexokinase into glucose 6-phosphate to discourage glucose efflux. SLC2A1-4 is upregulated in multiple cancer types (e.g., pancreatic, lung, and prostate), so there is significant interest in developing therapeutics targeting these transporters (Adekola et al., 2012; Holman, 2020; Pliszka and Szablewski, 2021). The SLC5 family are sodium symporters that can import their substrates, including glucose, against their gradient due to the Na+ electrochemical gradient. Members of the family, such as SLC5A2 and SLC5A5, are upregulated in certain cancer types (Gyimesi et al., 2020).
In concordance with the dysregulation of SLC2/5 family carbohydrate transporters and regulatory miRNAs in cancer cells, some miRNAs have been demonstrated to govern the expression of SLC2/5 genes (Table 1), in which the facilitative uniporter SLC2A1/GLUT1 is particularly well studied. SLC2A1 is present in most cells, with the highest expression in erythrocyte membranes (Mueckler and Thorens, 2013). Many SLC2A1 targeting miRNAs are downregulated in cancer cells, such as miR-148b in gastric cancer, miR-218 in bladder cancer, and miR-328 in colon cancer (Ding et al., 2017; Li et al., 2017; Santasusagna et al., 2018). Both miR-22 and miR-140-5p have been reported to target SLC2A1 in breast cancer (Chen B. et al., 2015; He et al., 2019). In prostate cancer, miR-378a and miR-132 can modulate SLC2A1 expression (Qu et al., 2016; Cannistraci et al., 2022). The oncogenic long ncRNA plasmacytoma variant translocation 1 was shown to sponge miR-378c in lung adenocarcinoma and prevent miR-378c binding to SLC2A1, resulting in an increase in SLC2A1 levels (Xia et al., 2021). MiR-378a-3p was found to be downregulated in prostate cancer, and miR-378a-3p was revealed to reduce SLC2A1 expression to interfere with glycolytic pathway and cell proliferation (Cannistraci et al., 2022).
Lactate Transporters and MicroRNA Regulation
The SLC16A subfamily proteins are monocarboxylate transporters (MCTs) that use the proton gradient to transport endobiotic (e.g., lactate and pyruvate) and xenobiotic (e.g., γ-hydroxybutyric acid) (Morris and Felmlee, 2008). Of particular interest are SLC16A1 (MCT1), which is present in the plasma membrane of most cells, and SLC16A3 (MCT4), which is more highly expressed in glycolytic cells such as skeletal muscle cells (Price et al., 1998; Payen et al., 2020). SLC16A1 may also be expressed in the nuclear, sarcolemma, and mitochondrial membrane (Park et al., 2018). SLC16A1 and SLC16A3 are overexpressed and associated with poor prognosis in cancers, such as pancreatic cancer (Yu S. et al., 2020). Lactate can be used by oxidative cancer cells as a signaling molecule with dysregulated SLC16A proteins (Payen et al., 2020). During metabolic symbiosis, SLC16A1 is involved in the influx of lactic acid into oxidative cancer cells, while SLC16A3 is involved in the efflux of lactate from glycolytic cancer cells (Sonveaux et al., 2008; Payen et al., 2020). SLC16A1 inhibition blocks tumor growth by increasing glucose use by oxidative cancer cells, which results in hypoxic cancer cell death due to glucose starvation (Sonveaux et al., 2008; Wang et al., 2022). Additionally, high levels of extracellular lactate will inhibit lactate efflux, which can block T-lymphocytes from proliferating and contribute to immune resistance (Fischer et al., 2007).
Some miRNAs have shown to modulate SLC16A1 expression and influence lactate transport in cancer cells (Table 2). In medulloblastomas, miR-124 was found to directly regulate SLC16A1, but miR-124 was downregulated in most tumors, which lead to an increase in SLC16A1 (Li et al., 2009). In triple negative breast cancer, miR-342-3p was found to directly reduce SLC16A1; as miR-342-3p was downregulated due to a lack of estrogen receptor, SLC16A1 levels were shown to be increased (Romero-Cordoba et al., 2018). Transfection with miR-342-3p mimic reduced SLC16A1 protein levels in breast cancer cells, and miR-342-3p overexpression reduced lactate influx in MDAMB468 cells, resulting in the accumulation of extracellular lactate, which disrupted metabolic symbiosis and increased competition for glucose (Romero-Cordoba et al., 2018) (Table 2).
Amino Acid Transporters and MicroRNA Regulation
SLC1A5
SLC1A5 (alanine, serine, cysteine transporter 2 or ASCT2) is a Na+-dependent antiporter in the plasma membrane involved in the exchange of neutral amino acids, such as glutamine. It has been known since the 1950s that HeLa cells use significantly more glutamine than other AAs (Eagle, 1955). Once imported into the cell, glutamine is converted to glutamate, which encourages the continued influx of glutamine and prevents efflux. SLC1A5 is commonly expressed in lung, skeletal muscle, large intestine, kidney, and adipose tissue (Kanai et al., 2013). SLC1A5 levels are increased in tissues that need high levels of glutamine for metabolism, as well as in cancer cells, including cancer from tissues that normally lack SLC1A5 (Lopes et al., 2021).
MiR-137 has been shown to directly bind to SLC1A5 in melanoma, which suppresses ferroptosis through the downregulation of SLC1A5 (Luo et al., 2018) (Table 3). MiR-137 is commonly downregulated in melanoma, so these cells might be sensitive to ferroptosis (Luo et al., 2018). Indeed, miR-137 was shown to regulate apoptosis, autophagy, and ferroptosis (Luo et al., 2018). In addition, miR-107, miR-149-5p, and miR-924 were shown to regulate SLC1A5 in esophageal cancer, breast cancer, and non-small-cell lung cancer cells respectively; however, they were sponged by circular RNA to prevent downregulation of SLC1A5 (Chang et al., 2021; Wang J. et al., 2021; Liu et al., 2022) (Table 3). There is also an interest in combining the inhibition of SLC1A5 and SLC7A5, since both are involved in the transport of crucial AAs (Kanai, 2022).
SLC7A5
SLC7A5 (L-type amino acid transporter 1 or LAT1) forms heterodimer with SLC3A2 to function as a Na+ independent, large neutral amino acid antiporter (Kanai et al., 1998; Mastroberardino et al., 1998) that is commonly overexpressed in cancer cells (Kanai, 2022). SLC7A5/SLC3A2 is normally expressed in the plasma membrane of testis, bone marrow, brain, and placenta (Scalise et al., 2018). SLC7A5 was also found to be expressed in the cytoplasm of liver and skeletal muscle, as well as in the lysosomal membrane for mTORC1 activation (Milkereit et al., 2015; Kanai, 2022). SLC7A5-mediated import of leucine, as well as other essential AAs, is crucial for cancer cells. It has been proposed in the past that the glutamine imported through SLC1A5 may be used for SLC7A5 efflux; however, this has been challenged due to the low affinity of SLC7A5 for glutamine (Scalise et al., 2017).
MiR-126 has been identified as a direct regulator of SLC7A5 in both gastric and lung cancer, while miR-126 is downregulated in gastric cancer and can function as a tumor suppressor (Feng et al., 2010) (Table 3). While SLC7A5 is upregulated in gastric cancer cells, miR-126 directly targets SLC7A5 and miR-126 overexpression can inhibit cancer cell proliferation (Wang et al., 2015). Similarly, miR-126 is downregulated in small cell lung cancer, and miR-126 directly regulates SLC7A5 expression (Miko et al., 2011). Long ncRNA plasmacytoma variant translocation 1–5 has been shown to act as a sponge for miR-126 in lung cancer cell lines, which prevents miR-126 from regulating SLC7A5 (Li H. et al., 2018). In esophageal squamous cell carcinoma, elevated miR-6775-3p levels were associated with better prognosis, and overexpression of miR-6775-3p blocked tumor growth and metastasis in xenograft mouse model (Meng et al., 2018). It was also found that p53 directly upregulates miR-6775-3p and SLC7A5, then miR-6775-3p downregulates SLC7A5 as well as the MAGE-A family (Meng et al., 2018). In addition, SLC7A5 has been verified as a direct target for miR-328-3p, whereas the reduction of LAT1 protein levels by miR-328-3p in osteosarcoma cells does not alter the overall levels of AAs (Yi et al., 2020), highlighting the importance to examine the impact on substrate transport or balance and indicating the presence of complex regulatory network behind AA homeostasis. Nevertheless, miR-328-3p also modulates the expression of other cancer-related genes, including SLC2A1, and synergistically inhibits cancer cell proliferation with chemotherapeutics (Yi et al., 2020) (Table 3).
SLC7A11
System Xc− is a heterodimeric cystine/glutamate antiporter made of the subunits SLC7A11 and SLC3A2, which is normally primarily expressed in the plasma membrane of the central nervous system (Lewerenz et al., 2013). System Xc− is upregulated in cancer cells to increase the production of glutathione from cystine, resulting in the prevention of ferroptosis (Liu et al., 2021). Without glutathione, glutathione peroxidase 4 is inactive, which causes an increase in intracellular lipid peroxidation, eventually culminating in ferroptosis (Han et al., 2020).
Multiple miRNAs have been identified as direct regulators of SLC7A11 (Table 3). MiR-375 is downregulated in oral squamous cell carcinoma, and treatment with miR-375 mimics reduced SLC7A11 levels and acted as a tumor suppressor (Wu et al., 2017). Similarly, miR-139-5p is reduced in pancreatic carcinoma, and the overexpression of miR-139-5p reduces SLC7A11 expression and also suppresses phosphatidylinositol 3-kinase and phosphorylated protein kinase B (Zhu et al., 2020). MiR-26b is downregulated in breast cancer cells, and treatment with miR-26b mimics downregulates SLC7A11 and causes apoptosis (Liu et al., 2011). MiR-27a was found to be downregulated in cisplatin-resistant bladder cancer cells, and overexpression of miR-27a repressed SLC7A11 expression and increased cell sensitivity to cisplatin (Drayton et al., 2014). MiR-382-5p was found to directly interact with SLC7A11 in ovarian and breast cancer cells, whereas miR-382-5p was downregulated and SLC7A11 was upregulated (Sun et al., 2021). Interestingly, treatment with lidocaine increased miR-382-5p, which resulted in ferroptosis due to a decrease in SLC7A11 (Sun et al., 2021). Some circular RNAs have also been found to sponge miRNAs to prevent their regulation of SLC7A11 in carcinoma cells. MiR-557 was sponged by circular RNA eukaryotic translation initiation factor 6 (circEIF6) in pancreatic cancer cells, miR-876-5p was sponged by circRNA CDR1 antisense RNA (circCDR1as) in oral squamous cell carcinoma cells, and miR-1261 was sponged by the circular RNA circ0097009 in hepatocellular cancer cells, all of which were linked to the upregulation of SLC7A11 (Cui et al., 2021; Lyu et al., 2021; Zhang T. et al., 2021) (Table 3).
Drug-Transporting SLCs and MicroRNA Regulation
Some SLC transporters, such as organic anion- or cation-transporting polypeptides (OATs, OATPs, or OCTs), are crucial for the uptake or efflux of xenobiotic medications (e.g., statins and chemotherapeutics) and toxins (e.g., microcystin and phalloidin), besides endobiotic metabolites (Roth et al., 2012; Zhou et al., 2017; Brecht et al., 2020). For instance, the SLC28 (concentrative nucleoside transporters or CNT) and SLC29 (equilibrative nucleoside transporters or ENT) families are involved in the influx of nucleoside and nucleobase drugs, such as clofarabine and gemcitabine (Young et al., 2013). The SLCO (OATP), SLC22A (OCT OAT, etc.), and SLC15A (peptide transporter or PEPT) families are key for the uptake of some statins (e.g., pravastatin and pitavastatin) and anticancer drugs (e.g., paclitaxel and doxorubicin), which may have significant impact on pharmacokinetics (Zhou et al., 2017; Brecht et al., 2020). Among them, SLCO1B3 is present in the basolateral membrane of hepatocytes and transports the chemotherapeutics docetaxel, paclitaxel, doxorubicin, cisplatin, carboplatin, and oxaliplatin (Schulte and Ho, 2019).
Dysregulation of both miRNA and solute carrier transporters have been shown to be associated with chemoresistance (Si et al., 2019; Sun et al., 2020; Rashid et al., 2021). MiR-579-3p was found to directly downregulate SLCO1B3 in pancreatic cancer cells, whereas the androgen biosynthesis inhibitor abiraterone downregulates miR-579-3p (Barbier et al., 2021) (Table 4). SLCO1B3 is also involved in the influx of testosterone, so the increase in SLCO1B3 is associated with resistance to androgen deprivation therapy (Barbier et al., 2021). In acute lymphoblastic leukemia, miR-595 downregulates the methotrexate transporter SLC19A1 with rs1051296 G > T polymorphism, and this decrease in SLC19A1 causes reduced methotrexate influx and sensitivity (Wang et al., 2018). This study shows that a single nucleotide polymorphism may alter the miRNA regulation of a key chemotherapeutic transporter; however, this was tested in a single cell line, so validation remains to be done (Wang et al., 2018). Further experimental determination and validation are required to delineate the influence of the miRNA-controlled regulation of SLC transporters on drug exposure and therapeutic outcomes.
Other SLC Transporters Regulated by MicroRNAs
Some miRNAs have been revealed to regulate key vitamin- and neurotransmitter-transporting SLCs in models outside of cancer (Table 5). MiR-103a was found to directly downregulate the ascorbic acid transporter SLC23A1, and treatment with miR-103a decreased the influx of ascorbic acid, also known as vitamin C, in the intestinal epithelial cell line Caco-2 (Subramanian et al., 2019). Additionally, miR-141 and miR-200a directly target the ascorbic acid transporter SLC23A2 in mouse bone marrow stromal cells, which reduced osteogenic differentiation (Sangani et al., 2015). In another study that used Caco-2 and HuTu-80 cells as well as mouse intestinal enteroids, miR-423-5p mimic downregulates the riboflavin transporter SLC52A3 and causes a decrease in riboflavin influx (Lakhan et al., 2017). Mouse mmu-miR-16-5p was found to directly downregulate the serotonin transporter SLC6A4, and the antidepressant fluoxetine, a selective serotonin reuptake inhibitor, was shown to upregulate mmu-miR-16-5p (Baudry et al., 2010). This suggests that one mechanism of decreasing serotonin influx by fluoxetine is through the miR-16-5p-mediated downregulation of serotonin transporter (Baudry et al., 2010).
MicroRNA-Based Therapies
With improved understanding of miRNA biology and regulatory approval of small interfering RNA (siRNA) medications (Yu A.-M. et al., 2020; Moumné et al., 2022; Yu and Tu, 2022), there is great interest in the development of miRNA-based therapeutics. The first siRNA medication, patisiran, was approved by the United States (US) Food and Drug Administration (FDA) in 2018 for the treatment of transthyretin amyloidosis by targeting the 3′UTR of the transthyretin mRNA (Setten et al., 2019). The second siRNA drug, givosiran, was approved by the FDA in 2019 for the treatment of acute intermittent porphyria (De Paula Brandão et al., 2020). Lumasiran was approved by the FDA in 2020 for the treatment of primary hyperoxaluria type 1 by targeting the 3′UTR of hydroxyacid oxidase 1 mRNA (Hulton, 2021). Inclisiran was approved in December 2021 for the treatment of heterozygous familial hypercholesterolemia or clinical atherosclerotic cardiovascular disease by reducing low-density lipoprotein cholesterol via the regulation of proprotein convertase subtilisin–kexin type 9, in combination with statin therapy (Raal et al., 2020). It is noteworthy that all of the four siRNA drugs approved by the FDA act on hepatic targets, and among them, three (patisiran, lumasiran, and inclisiran) follow the miRNA mechanism of action to target the 3′UTR (Zhang M. M. et al., 2021; Yu and Tu, 2022). These drugs have proved the concept of RNA interference (RNAi) therapy, including genome-derived miRNAs as therapeutics or targets (Yu A.-M. et al., 2020).
Depending on the miRNA function and dysregulation profile, strategies may employ to either inhibit or restore the miRNA expression or function (Hanna et al., 2019; Yu A.-M. et al., 2020). To inhibit miRNA function, one may use miRNA inhibitors or antagomirs, which are antisense oligonucleotides that bind to miRNA to prevent miRNA from repressing their targets and miRNA competitors, or block-mirs, which prevent the recognition of miRNA binding sites on target mRNA (Setten et al., 2019). There is also a growing interest in developing small-molecule miRNA inhibitors (Ursu et al., 2020; Yu A.-M. et al., 2020; Fu et al., 2021). On the other hand, chemically synthesized miRNA mimics are commonly used to restore miRNA functions in cells. An alternative approach to miRNA mimics has been developed to produce bioengineered RNA molecules (Chen Q.-X. et al., 2015; Ho et al., 2018; Li P.-C. et al., 2018; Deng et al., 2021; Li P.-C. et al., 2021; Tu et al., 2021). The challenges of developing miRNA therapeutics have been discussed in recent articles (Segal and Slack, 2020; Kara et al., 2022; Yu and Tu, 2022), which include minimizing the degradation from nucleases, improving target cell uptake, and avoiding off-target or unwanted side effects.
MRX34, a miR-34a mimic that is effective to suppress tumor progression in animal models through multiple mechanisms including the interference with cancer metabolism via regulating SLC2A1 expression (He et al., 2019; Hong M. et al., 2020), was the first anticancer miRNA to reach phase I clinical trials, but the trial ended in 2016 due to the occurrence of severe immune-related adverse events and even mortality (Hong D. S. et al., 2020). While this trial demonstrates the need for cancer cell-targeted delivery systems since the liposomal nanoparticle SMARTICLE used in the clinical trial is not a cancer cell-specific delivery system (Li W. et al., 2021), caution is advised to select the right molecular entity at the right dose and administer at the right time to achieve efficacious and safe therapy. Nevertheless, there are multiple other therapeutic miRNAs under phase II clinical trials, such as the miR-126 mimic TargomiR and anti-miR-155 Cobomarsen (Kara et al., 2022). Among them, the interactions between tumor-suppressive miRNAs (e.g., miR-126) and SLC transporters (e.g., SLC7A5) not only support the concept of targeting critical SLC transporters for the control of diseases (e.g., cancer) (Zhang et al., 2019; Wang et al., 2020) but also develop effective miRNA medications that may act on multiple therapeutic targets including SLC transporters.
Conclusion and Perspectives
Our understanding of nutrient metabolism has rapidly developed since Otto Warburg observed aerobic glycolysis in cancer cells in the 1920s. Metabolic reprogramming has been recognized as a hallmark of cancer, and there is ongoing interest in identifying the determinant factors contributing to cancer metabolism, as well as developing respective therapeutic strategies. SLC transporters are commonly dysregulated to support the increased demand for nutrients and metabolites. While some SLCs may not be regulated at the posttranscriptional levels, many studies have demonstrated the direct regulation of SLCs by miRNAs, offering insights into the causes of the altered expression of SLCs in cancer cells. As SLCs are known to transport many critical nutrients as well as endobiotic metabolites, the impact of miRNA–SLC signaling on cancer metabolism cannot be underestimated. Therefore, the intervention of critical miRNA–SLC pathways underlying dysregulated cell metabolism represents a new strategy to control related diseases, including cancer, which may be witnessed in future clinical investigations or even practice. Furthermore, less is known regarding the potential roles of miRNA regulation on drug-transporting SLCs in pharmacotherapy, including possible effects on drug exposure, efficacy, and safety. Therefore, more research in these areas is expected to improve our knowledge on miRNA–SLC interactions in drug development and clinical therapy. Additionally, there are still many orphan SLCs with unknown functions and substrates. Both miRNA and SLCs have a multitude of members, with the well-known receiving a disproportionate amount of attention. It would be beneficial to invest in researching orphan transporters to uncover new interactions and importance in cell metabolism as well as implication to diseases.
With an increased understanding in how some miRNAs regulate cancer-related SLCs, new therapeutic approaches may be developed to either restore or inhibit miRNA functions to control tumor progression and metastasis. Multiple miRNA-based anticancer therapeutics have entered clinical trials. However, the failure of MRX34 due to severe and even fatal adverse reactions highlights the needs for selecting the right therapeutic molecules as well as delivery systems to achieve the desired efficacy and safety. Furthermore, a single miRNA may have multiple targets in the cells, so the full effects of therapeutic miRNA should be carefully defined and considered. Some miRNAs clearly have crucial roles in the regulation of cancer progression, in part, through the direct regulation of key SLC transporters, and the development of miRNA-based therapies, monotherapy or combination with other means, has the potential to vastly improve cancer treatments.
Author Contributions
Both CY and A‐MY contributed to literature research, analysis, writing, and revisions.
Funding
This study was supported by the National Institute of General Medical Sciences (R35GM140835) and the National Cancer Institute (grant Nos. R01CA253230 and R01CA225958), National Institutes of Health.
Conflict of Interest
The authors declare that the research was conducted in the absence of any commercial or financial relationships that could be construed as a potential conflict of interest.
Publisher’s Note
All claims expressed in this article are solely those of the authors and do not necessarily represent those of their affiliated organizations, or those of the publisher, the editors, and the reviewers. Any product that may be evaluated in this article, or claim that may be made by its manufacturer, is not guaranteed or endorsed by the publisher.
Abbreviations
AA, amino acid; circRNA, circular RNA; GLUT, glucose transporter; MCT, monocarboxylate transporters; MRE, microRNA response element; miRNA or miR, MicroRNA; ncRNA, noncoding RNA; PPP, pentose phosphate pathway; SLC, solute carrier; 3′UTR, 3′-untranslated region.
References
Adekola, K., Rosen, S. T., and Shanmugam, M. (2012). Glucose Transporters in Cancer Metabolism. Curr. Opin. Oncol. 24, 650–654. doi:10.1097/cco.0b013e328356da72
Ali Syeda, Z., Langden, S. S. S., Munkhzul, C., Lee, M., and Song, S. J. (2020). Regulatory Mechanism of MicroRNA Expression in Cancer. Int. J. Mol. Sci. 21, 1723. doi:10.3390/ijms21051723
Anbazhagan, A. N., Priyamvada, S., Borthakur, A., Saksena, S., Gill, R. K., Alrefai, W. A., et al. (2019). miR-125a-5p: a Novel Regulator of SLC26A6 Expression in Intestinal Epithelial Cells. Am. J. Physiology-Cell Physiology 317, C200–C208. doi:10.1152/ajpcell.00068.2019
Anbazhagan, A. N., Priyamvada, S., Kumar, A., Maher, D. B., Borthakur, A., Alrefai, W. A., et al. (2014). Translational Repression of SLC26A3 by miR-494 in Intestinal Epithelial Cells. Am. J. Physiology-Gastrointestinal Liver Physiology 306, G123–G131. doi:10.1152/ajpgi.00222.2013
Ancey, P. B., Contat, C., and Meylan, E. (2018). Glucose Transporters in Cancer - from Tumor Cells to the Tumor Microenvironment. FEBS J. 285, 2926–2943. doi:10.1111/febs.14577
Anfossi, S., Fu, X., Nagvekar, R., and Calin, G. A. (2018). MicroRNAs, Regulatory Messengers inside and outside Cancer Cells. Adv. Exp. Med. Biol. 1056, 87–108. doi:10.1007/978-3-319-74470-4_6
Bai, X., Moraes, T. F., and Reithmeier, R. A. F. (2017). Structural Biology of Solute Carrier (SLC) Membrane Transport Proteins. Mol. Membr. Biol. 34, 1–32. doi:10.1080/09687688.2018.1448123
Barbier, R. H., Mccrea, E. M., Lee, K. Y., Strope, J. D., Risdon, E. N., Price, D. K., et al. (2021). Abiraterone Induces SLCO1B3 Expression in Prostate Cancer via microRNA-579-3p. Sci. Rep. 11, 10765. doi:10.1038/s41598-021-90143-4
Baudry, A., Mouillet-Richard, S., Schneider, B., Launay, J.-M., and Kellermann, O. (2010). miR-16 Targets the Serotonin Transporter: a New Facet for Adaptive Responses to Antidepressants. Science 329, 1537–1541. doi:10.1126/science.1193692
Bhattacharyya, S. N., Habermacher, R., Martine, U., Closs, E. I., and Filipowicz, W. (2006). Relief of microRNA-Mediated Translational Repression in Human Cells Subjected to Stress. Cell 125, 1111–1124. doi:10.1016/j.cell.2006.04.031
Brecht, K., Schäfer, A. M., and Meyer Zu Schwabedissen, H. E. (2020). Uptake Transporters of the SLC21, SLC22A, and SLC15A Families in Anticancer Therapy-Modulators of Cellular Entry or Pharmacokinetics? Cancers (Basel) 12, 2263. doi:10.3390/cancers12082263
Brennecke, J., Stark, A., Russell, R. B., and Cohen, S. M. (2005). Principles of microRNA-Target Recognition. PLoS Biol. 3, e85. doi:10.1371/journal.pbio.0030085
Bröer, A., Rahimi, F., and Bröer, S. (2016). Deletion of Amino Acid Transporter ASCT2 (SLC1A5) Reveals an Essential Role for Transporters SNAT1 (SLC38A1) and SNAT2 (SLC38A2) to Sustain Glutaminolysis in Cancer Cells. J. Biol. Chem. 291, 13194–13205. doi:10.1074/jbc.m115.700534
Bröer, S. (2020). Amino Acid Transporters as Targets for Cancer Therapy: Why, where, when, and How. Int. J. Mol. Sci. 21, 6156. doi:10.3390/ijms21176156
Calin, G. A., Sevignani, C., Dumitru, C. D., Hyslop, T., Noch, E., Yendamuri, S., et al. (2004). Human microRNA Genes Are Frequently Located at Fragile Sites and Genomic Regions Involved in Cancers. Proc. Natl. Acad. Sci. U.S.A. 101, 2999–3004. doi:10.1073/pnas.0307323101
Cannistraci, A., Hascoet, P., Ali, A., Mundra, P., Clarke, N. W., Pavet, V., et al. (2022). MiR-378a Inhibits Glucose Metabolism by Suppressing GLUT1 in Prostate Cancer. Oncogene 41 (10), 1445–1455. doi:10.1038/s41388-022-02178-0
Capri, M., Olivieri, F., Lanzarini, C., Remondini, D., Borelli, V., Lazzarini, R., et al. (2017). Identification of miR-31-5p, miR-141-3p, miR-200c-3p, and GLT1 as Human Liver Aging Markers Sensitive to Donor-Recipient Age-Mismatch in Transplants. Aging Cell 16, 262–272. doi:10.1111/acel.12549
César-Razquin, A., Snijder, B., Frappier-Brinton, T., Isserlin, R., Gyimesi, G., Bai, X., et al. (2015). A Call for Systematic Research on Solute Carriers. Cell 162, 478–487. doi:10.1016/j.cell.2015.07.022
Chang, J., Nicolas, E., Marks, D., Sander, C., Lerro, A., Buendia, M. A., et al. (2004). miR-122, a Mammalian Liver-specific microRNA, Is Processed from Hcr mRNA and MayDownregulate the High Affinity Cationic Amino Acid Transporter CAT-1. RNA Biol. 1, 106–113. doi:10.4161/rna.1.2.1066
Chang, Z., Fu, Y., Jia, Y., Gao, M., Song, L., Zhang, W., et al. (2021). Circ-SFMBT2 Drives the Malignant Phenotypes of Esophageal Cancer by the miR-107-dependent Regulation of SLC1A5. Cancer Cell Int. 21, 495. doi:10.1186/s12935-021-02156-8
Chen, B., Tang, H., Liu, X., Liu, P., Yang, L., Xie, X., et al. (2015). miR-22 as a Prognostic Factor Targets Glucose Transporter Protein Type 1 in Breast Cancer. Cancer Lett. 356, 410–417. doi:10.1016/j.canlet.2014.09.028
Chen, D., Wang, H., Chen, J., Li, Z., Li, S., Hu, Z., et al. (2018). MicroRNA-129-5p Regulates Glycolysis and Cell Proliferation by Targeting the Glucose Transporter SLC2A3 in Gastric Cancer Cells. Front. Pharmacol. 9, 502. doi:10.3389/fphar.2018.00502
Chen, G., Feng, Y., Li, X., Jiang, Z., Bei, B., Zhang, L., et al. (2019). Post-transcriptional Gene Regulation in Colitis Associated Cancer. Front. Genet. 10, 585. doi:10.3389/fgene.2019.00585
Chen, Q.-X., Wang, W.-P., Zeng, S., Urayama, S., and Yu, A.-M. (2015). A General Approach to High-Yield Biosynthesis of Chimeric RNAs Bearing Various Types of Functional Small RNAs for Broad Applications. Nucleic Acids Res. 43, 3857–3869. doi:10.1093/nar/gkv228
Chuang, T. Y., Wu, H. L., Chen, C. C., Gamboa, G. M., Layman, L. C., Diamond, M. P., et al. (2015). MicroRNA-223 Expression Is Upregulated in Insulin Resistant Human Adipose Tissue. J. Diabetes Res. 2015, 943659. doi:10.1155/2015/943659
Cui, L., Huang, C., and Zhou, D. (Online ahead of print). Overexpression of circCDR1as Drives Oral Squamous Cell Carcinoma Progression. Oral Dis. doi:10.1111/odi.14085
Czuba, L. C., Hillgren, K. M., and Swaan, P. W. (2018). Post-translational Modifications of Transporters. Pharmacol. Ther. 192, 88–99. doi:10.1016/j.pharmthera.2018.06.013
Dai, D.-W., Lu, Q., Wang, L.-X., Zhao, W.-Y., Cao, Y.-Q., Li, Y.-N., et al. (2013). Decreased miR-106a Inhibits Glioma Cell Glucose Uptake and Proliferation by Targeting SLC2A3 in GBM. BMC Cancer 13, 478. doi:10.1186/1471-2407-13-478
Dai, X., Chen, X., Chen, Q., Shi, L., Liang, H., Zhou, Z., et al. (2015). MicroRNA-193a-3p Reduces Intestinal Inflammation in Response to Microbiota via Down-Regulation of Colonic PepT1. J. Biol. Chem. 290, 16099–16115. doi:10.1074/jbc.m115.659318
Dalmasso, G., Nguyen, H. T. T., Yan, Y., Laroui, H., Charania, M. A., Obertone, T. S., et al. (2011). MicroRNA-92b Regulates Expression of the Oligopeptide Transporter PepT1 in Intestinal Epithelial Cells. Am. J. Physiology-Gastrointestinal Liver Physiology 300, G52–G59. doi:10.1152/ajpgi.00394.2010
De Paula Brandão, P. R., Titze-De-Almeida, S. S., and Titze-De-Almeida, R. (2020). Leading RNA Interference Therapeutics Part 2: Silencing Delta-Aminolevulinic Acid Synthase 1, with a Focus on Givosiran. Mol. Diagnosis Ther. 24, 61–68. doi:10.1007/s40291-019-00438-6
Deberardinis, R. J., Mancuso, A., Daikhin, E., Nissim, I., Yudkoff, M., Wehrli, S., et al. (2007). Beyond Aerobic Glycolysis: Transformed Cells Can Engage in Glutamine Metabolism that Exceeds the Requirement for Protein and Nucleotide Synthesis. Proc. Natl. Acad. Sci. U.S.A. 104, 19345–19350. doi:10.1073/pnas.0709747104
Deng, L., Petrek, H., Tu, M.-J., Batra, N., Yu, A.-X., and Yu, A.-M. (2021). Bioengineered miR-124-3p Prodrug Selectively Alters the Proteome of Human Carcinoma Cells to Control Multiple Cellular Components and Lung Metastasis In Vivo. Acta Pharm. Sin. B 11, 3950–3965. doi:10.1016/j.apsb.2021.07.027
Denli, A. M., Tops, B. B. J., Plasterk, R. H. A., Ketting, R. F., and Hannon, G. J. (2004). Processing of Primary microRNAs by the Microprocessor Complex. Nature 432, 231–235. doi:10.1038/nature03049
Ding, C., Ding, X., Zheng, J., Wang, B., Li, Y., Xiang, H., et al. (2020). miR-182-5p and miR-378a-3p Regulate Ferroptosis in I/R-induced Renal Injury. Cell Death Dis. 11, 929. doi:10.1038/s41419-020-03135-z
Ding, X., Liu, J., Liu, T., Ma, Z., Wen, D., and Zhu, J. (2017). miR-148b Inhibits Glycolysis in Gastric Cancer through Targeting SLC2A1. Cancer Med. 6, 1301–1310. doi:10.1002/cam4.1008
Dragomir, M. P., Knutsen, E., and Calin, G. A. (2021). Classical and Noncanonical Functions of miRNAs in Cancers. Trends Genet. 38 (4), 379–394. doi:10.1016/j.tig.2021.10.002
Drayton, R. M., Dudziec, E., Peter, S., Bertz, S., Hartmann, A., Bryant, H. E., et al. (2014). Reduced Expression of miRNA-27a Modulates Cisplatin Resistance in Bladder Cancer by Targeting the Cystine/glutamate Exchanger SLC7A11. Clin. Cancer Res. 20, 1990–2000. doi:10.1158/1078-0432.ccr-13-2805
Eagle, H. (1955). The Minimum Vitamin Requirements of the L and HeLa Cells in Tissue Culture, the Production of Specific Vitamin Deficiencies, and Their Cure. J. Exp. Med. 102, 595–600. doi:10.1084/jem.102.5.595
Fan, Y., Zhou, Y., Zhou, X., Sun, F., Gao, B., Wan, M., et al. (2015). MicroRNA 224 Regulates Ion Transporter Expression in Ameloblasts to Coordinate Enamel Mineralization. Mol. Cell Biol. 35, 2875–2890. doi:10.1128/mcb.01266-14
Fei, X., Qi, M., Wu, B., Song, Y., Wang, Y., and Li, T. (2012). MicroRNA-195-5p Suppresses Glucose Uptake and Proliferation of Human Bladder Cancer T24 Cells by regulatingGLUT3expression. FEBS Lett. 586, 392–397. doi:10.1016/j.febslet.2012.01.006
Felmlee, M. A., Jones, R. S., Rodriguez-Cruz, V., Follman, K. E., and Morris, M. E. (2020). Monocarboxylate Transporters (SLC16): Function, Regulation, and Role in Health and Disease. Pharmacol. Rev. 72, 466–485. doi:10.1124/pr.119.018762
Feng, R., Chen, X., Yu, Y., Su, L., Yu, B., Li, J., et al. (2010). miR-126 Functions as a Tumour Suppressor in Human Gastric Cancer. Cancer Lett. 298, 50–63. doi:10.1016/j.canlet.2010.06.004
Fischer, K., Hoffmann, P., Voelkl, S., Meidenbauer, N., Ammer, J., Edinger, M., et al. (2007). Inhibitory Effect of Tumor Cell-Derived Lactic Acid on Human T Cells. Blood 109, 3812–3819. doi:10.1182/blood-2006-07-035972
Friedman, R. C., Farh, K. K.-H., Burge, C. B., and Bartel, D. P. (2009). Most Mammalian mRNAs Are Conserved Targets of microRNAs. Genome Res. 19, 92–105. doi:10.1101/gr.082701.108
Fu, Z., Wang, L., Li, S., Chen, F., Au-Yeung, K. K.-W., and Shi, C. (2021). MicroRNA as an Important Target for Anticancer Drug Development. Front. Pharmacol. 12, 736323. doi:10.3389/fphar.2021.736323
Gao, W., Bi, Y., Ding, L., Zhu, W., and Ye, M. (2017). SSa Ameliorates the Glu Uptaking Capacity of Astrocytes in Epilepsy via AP-1/miR-155/GLAST. Biochem. Biophysical Res. Commun. 493, 1329–1335. doi:10.1016/j.bbrc.2017.09.139
Gyimesi, G., Pujol-Giménez, J., Kanai, Y., and Hediger, M. A. (2020). Sodium-coupled Glucose Transport, the SLC5 Family, and Therapeutically Relevant Inhibitors: from Molecular Discovery to Clinical Application. Pflugers Arch. - Eur. J. Physiol. 472, 1177–1206. doi:10.1007/s00424-020-02433-x
Han, C., Liu, Y., Dai, R., Ismail, N., Su, W., and Li, B. (2020). Ferroptosis and its Potential Role in Human Diseases. Front. Pharmacol. 11, 239. doi:10.3389/fphar.2020.00239
Hanahan, D., and Weinberg, R. A. (2011). Hallmarks of Cancer: the Next Generation. Cell 144, 646–674. doi:10.1016/j.cell.2011.02.013
Hanna, J., Hossain, G. S., and Kocerha, J. (2019). The Potential for microRNA Therapeutics and Clinical Research. Front. Genet. 10, 478. doi:10.3389/fgene.2019.00478
Hata, A., and Kashima, R. (2016). Dysregulation of microRNA Biogenesis Machinery in Cancer. Crit. Rev. Biochem. Mol. Biol. 51, 121–134. doi:10.3109/10409238.2015.1117054
He, Y., Deng, F., Zhao, S., Zhong, S., Zhao, J., Wang, D., et al. (2019). Analysis of miRNA-mRNA Network Reveals miR-140-5p as a Suppressor of Breast Cancer Glycolysis via Targeting GLUT1. Epigenomics 11, 1021–1036. doi:10.2217/epi-2019-0072
Hediger, M. A., Clémençon, B., Burrier, R. E., and Bruford, E. A. (2013). The ABCs of Membrane Transporters in Health and Disease (SLC Series): Introduction. Mol. Aspects Med. 34, 95–107. doi:10.1016/j.mam.2012.12.009
Ho, P. Y., Duan, Z., Batra, N., Jilek, J. L., Tu, M.-J., Qiu, J.-X., et al. (2018). Bioengineered Noncoding RNAs Selectively Change Cellular miRNome Profiles for Cancer Therapy. J. Pharmacol. Exp. Ther. 365, 494–506. doi:10.1124/jpet.118.247775
Hoglund, P. J., Nordstrom, K. J. V., Schioth, H. B., and Fredriksson, R. (2011). The Solute Carrier Families Have a Remarkably Long Evolutionary History with the Majority of the Human Families Present before Divergence of Bilaterian Species. Mol. Biol. Evol. 28, 1531–1541. doi:10.1093/molbev/msq350
Holman, G. D. (2020). Structure, Function and Regulation of Mammalian Glucose Transporters of the SLC2 Family. Pflugers Arch. - Eur. J. Physiol. 472, 1155–1175. doi:10.1007/s00424-020-02411-3
Hommers, L. G., Richter, J., Yang, Y., Raab, A., Baumann, C., Lang, K., et al. (2018). A Functional Genetic Variation of SLC6A2 Repressor Hsa-miR-579-3p Upregulates Sympathetic Noradrenergic Processes of Fear and Anxiety. Transl. Psychiatry 8, 226. doi:10.1038/s41398-018-0278-4
Hong, D. S., Kang, Y.-K., Borad, M., Sachdev, J., Ejadi, S., Lim, H. Y., et al. (2020). Phase 1 Study of MRX34, a Liposomal miR-34a Mimic, in Patients with Advanced Solid Tumours. Br. J. Cancer 122, 1630–1637. doi:10.1038/s41416-020-0802-1
Hong, M., Zhang, X.-B., Xiang, F., Fei, X., Ouyang, X.-L., and Peng, X.-C. (2020). MiR-34a Suppresses Osteoblast Differentiation through Glycolysis Inhibition by Targeting Lactate Dehydrogenase-A (LDHA). Vitro Cell.Dev.Biol.-Animal 56, 480–487. doi:10.1007/s11626-020-00467-0
Hua, H., Kong, Q., Zhang, H., Wang, J., Luo, T., and Jiang, Y. (2019). Targeting mTOR for Cancer Therapy. J. Hematol. Oncol. 12, 71. doi:10.1186/s13045-019-0754-1
Hulton, S.-A. (2021). Lumasiran: Expanding the Treatment Options for Patients with Primary Hyperoxaluria Type 1. Expert Opin. Orphan Drugs 9, 189–198. doi:10.1080/21678707.2021.2003779
Jia, X., Wang, F., Han, Y., Geng, X., Li, M., Shi, Y., et al. (2016). miR-137 and miR-491 Negatively Regulate Dopamine Transporter Expression and Function in Neural Cells. Neurosci. Bull. 32, 512–522. doi:10.1007/s12264-016-0061-6
Kanai, Y. (2022). Amino Acid Transporter LAT1 (SLC7A5) as a Molecular Target for Cancer Diagnosis and Therapeutics. Pharmacol. Ther. 230, 107964. doi:10.1016/j.pharmthera.2021.107964
Kanai, Y., Clémençon, B., Simonin, A., Leuenberger, M., Lochner, M., Weisstanner, M., et al. (2013). The SLC1 High-Affinity Glutamate and Neutral Amino Acid Transporter Family. Mol. Aspects Med. 34, 108–120. doi:10.1016/j.mam.2013.01.001
Kanai, Y., Segawa, H., Miyamoto, K.-I., Uchino, H., Takeda, E., and Endou, H. (1998). Expression Cloning and Characterization of a Transporter for Large Neutral Amino Acids Activated by the Heavy Chain of 4F2 Antigen (CD98). J. Biol. Chem. 273, 23629–23632. doi:10.1074/jbc.273.37.23629
Kara, G., Calin, G. A., and Ozpolat, B. (2022). RNAi-based Therapeutics and Tumor Targeted Delivery in Cancer. Adv. Drug Deliv. Rev. 182, 114113. doi:10.1016/j.addr.2022.114113
King, B. C., Esguerra, J. L. S., Golec, E., Eliasson, L., Kemper, C., and Blom, A. M. (2016). CD46 Activation Regulates miR-150-Mediated Control of GLUT1 Expression and Cytokine Secretion in Human CD4+ T Cells. J. Immunol. 196, 1636–1645. doi:10.4049/jimmunol.1500516
Kinoshita, C., Aoyama, K., Matsumura, N., Kikuchi-Utsumi, K., Watabe, M., and Nakaki, T. (2014). Rhythmic Oscillations of the microRNA miR-96-5p Play a Neuroprotective Role by Indirectly Regulating Glutathione Levels. Nat. Commun. 5, 3823. doi:10.1038/ncomms4823
Kozal, K., Jóźwiak, P., and Krześlak, A. (2021). Contemporary Perspectives on the Warburg Effect Inhibition in Cancer Therapy. Cancer control. 28, 10732748211041243. doi:10.1177/10732748211041243
Krol, J., Busskamp, V., Markiewicz, I., Stadler, M. B., Ribi, S., Richter, J., et al. (2010). Characterizing Light-Regulated Retinal microRNAs Reveals Rapid Turnover as a Common Property of Neuronal microRNAs. Cell 141, 618–631. doi:10.1016/j.cell.2010.03.039
Lakhan, R., Subramanian, V. S., and Said, H. M. (2017). Role of MicroRNA-423-5p in Posttranscriptional Regulation of the Intestinal Riboflavin Transporter-3. Am. J. Physiology-Gastrointestinal Liver Physiology 313, G589–G598. doi:10.1152/ajpgi.00238.2017
Lee, R. C., Feinbaum, R. L., and Ambros, V. (1993). The C. elegans Heterochronic Gene Lin-4 Encodes Small RNAs with Antisense Complementarity to Lin-14. Cell 75, 843–854. doi:10.1016/0092-8674(93)90529-y
Lewerenz, J., Hewett, S. J., Huang, Y., Lambros, M., Gout, P. W., Kalivas, P. W., et al. (2013). The Cystine/Glutamate Antiporter System Xc−in Health and Disease: From Molecular Mechanisms to Novel Therapeutic Opportunities. Antioxidants Redox Signal. 18, 522–555. doi:10.1089/ars.2011.4391
Li, H., Chen, S., Liu, J., Guo, X., Xiang, X., Dong, T., et al. (2018). Long Non-coding RNA PVT1-5 Promotes Cell Proliferation by Regulating miR-126/SLC7A5 axis in Lung Cancer. Biochem. Biophysical Res. Commun. 495, 2350–2355. doi:10.1016/j.bbrc.2017.12.114
Li, J., Fu, F., Wan, X., Huang, S., Wu, D., and Li, Y. (2018). Up-regulated miR-29c Inhibits Cell Proliferation and Glycolysis by Inhibiting SLC2A3 Expression in Prostate Cancer. Gene 665, 26–34. doi:10.1016/j.gene.2018.04.086
Li, K. K. W., Pang, J. C.-S., Ching, A. K.-K., Wong, C. K., Kong, X., Wang, Y., et al. (2009). miR-124 Is Frequently Down-Regulated in Medulloblastoma and Is a Negative Regulator of SLC16A1. Hum. Pathol. 40, 1234–1243. doi:10.1016/j.humpath.2009.02.003
Li, P.-C., Tu, M.-J., Ho, P. Y., Batra, N., Tran, M. M. L., Qiu, J.-X., et al. (2021). In Vivo fermentation Production of Humanized Noncoding RNAs Carrying Payload miRNAs for Targeted Anticancer Therapy. Theranostics 11, 4858–4871. doi:10.7150/thno.56596
Li, P.-C., Tu, M.-J., Ho, P. Y., Jilek, J. L., Duan, Z., Zhang, Q.-Y., et al. (2018). Bioengineered NRF2-siRNA Is Effective to Interfere with NRF2 Pathways and Improve Chemosensitivity of Human Cancer Cells. Drug Metab. Dispos. 46, 2–10. doi:10.1124/dmd.117.078741
Li, P., Yang, X., Cheng, Y., Zhang, X., Yang, C., Deng, X., et al. (2017). MicroRNA-218 Increases the Sensitivity of Bladder Cancer to Cisplatin by Targeting Glut1. Cell Physiol. Biochem. 41, 921–932. doi:10.1159/000460505
Li, W., Wang, Y., Liu, R., Kasinski, A. L., Shen, H., Slack, F. J., et al. (2021). MicroRNA-34a: Potent Tumor Suppressor, Cancer Stem Cell Inhibitor, and Potential Anticancer Therapeutic. Front. Cell Dev. Biol. 9, 640587. doi:10.3389/fcell.2021.640587
Lian, J., Jing, Y., Dong, Q., Huan, L., Chen, D., Bao, C., et al. (2016). miR-192, a Prognostic Indicator, Targets the SLC39A6/SNAIL Pathway to Reduce Tumor Metastasis in Human Hepatocellular Carcinoma. Oncotarget 7, 2672–2683. doi:10.18632/oncotarget.6603
Lin, L., Yee, S. W., Kim, R. B., and Giacomini, K. M. (2015). SLC Transporters as Therapeutic Targets: Emerging Opportunities. Nat. Rev. Drug Discov. 14, 543–560. doi:10.1038/nrd4626
Liu, M.-R., Zhu, W.-T., and Pei, D.-S. (2021). System Xc−: a Key Regulatory Target of Ferroptosis in Cancer. Invest. New Drugs 39, 1123–1131. doi:10.1007/s10637-021-01070-0
Liu, X.-X., Li, X.-J., Zhang, B., Liang, Y.-J., Zhou, C.-X., Cao, D.-X., et al. (2011). MicroRNA-26b Is Underexpressed in Human Breast Cancer and Induces Cell Apoptosis by Targeting SLC7A11. FEBS Lett. 585, 1363–1367. doi:10.1016/j.febslet.2011.04.018
Liu, X., Olszewski, K., Zhang, Y., Lim, E. W., Shi, J., Zhang, X., et al. (2020). Cystine Transporter Regulation of Pentose Phosphate Pathway Dependency and Disulfide Stress Exposes a Targetable Metabolic Vulnerability in Cancer. Nat. Cell Biol. 22, 476–486. doi:10.1038/s41556-020-0496-x
Liu, Y., Wang, S., Pan, S., Yan, Q., Li, Y., and Zhao, Y. (2022). Circ_0000463 Contributes to the Progression and Glutamine Metabolism of Non-small-cell Lung Cancer by Targeting miR-924/SLC1A5 Signaling. J. Clin. Lab. Anal. 36, e24116. doi:10.1002/jcla.24116
Locasale, J. W., Grassian, A. R., Melman, T., Lyssiotis, C. A., Mattaini, K. R., Bass, A. J., et al. (2011). Phosphoglycerate Dehydrogenase Diverts Glycolytic Flux and Contributes to Oncogenesis. Nat. Genet. 43, 869–874. doi:10.1038/ng.890
Locasale, J. W. (2013). Serine, glycine and One-Carbon Units: Cancer Metabolism in Full Circle. Nat. Rev. Cancer 13, 572–583. doi:10.1038/nrc3557
Lopes, C., Pereira, C., and Medeiros, R. (2021). ASCT2 and LAT1 Contribution to the Hallmarks of Cancer: from a Molecular Perspective to Clinical Translation. Cancers 13, 203. doi:10.3390/cancers13020203
Lozada-Delgado, E. L., Grafals-Ruiz, N., Miranda-Roman, M. A., Santana-Rivera, Y., Valiyeva, F., Rivera-Diaz, M., et al. (2018). Targeting MicroRNA-143 Leads to Inhibition of Glioblastoma Tumor Progression. Cancers (Basel) 10, 382. doi:10.3390/cancers10100382
Lu, J., Getz, G., Miska, E. A., Alvarez-Saavedra, E., Lamb, J., Peck, D., et al. (2005). MicroRNA Expression Profiles Classify Human Cancers. Nature 435, 834–838. doi:10.1038/nature03702
Lu, X., Kang, N., Ling, X., Pan, M., Du, W., and Gao, S. (2021). MiR-27a-3p Promotes Non-small Cell Lung Cancer through SLC7A11-Mediated-Ferroptosis. Front. Oncol. 11, 759346. doi:10.3389/fonc.2021.759346
Luo, M., Wu, L., Zhang, K., Wang, H., Zhang, T., Gutierrez, L., et al. (2018). miR-137 Regulates Ferroptosis by Targeting Glutamine Transporter SLC1A5 in Melanoma. Cell Death Differ. 25, 1457–1472. doi:10.1038/s41418-017-0053-8
Lyu, N., Zeng, Y., Kong, Y., Chen, Q., Deng, H., Ou, S., et al. (2021). Ferroptosis Is Involved in the Progression of Hepatocellular Carcinoma through the circ0097009/miR-1261/SLC7A11 axis. Ann. Transl. Med. 9, 675. doi:10.21037/atm-21-997
Maolakuerban, N., Azhati, B., Tusong, H., Abula, A., Yasheng, A., and Xireyazidan, A. (2018). MiR-200c-3p Inhibits Cell Migration and Invasion of Clear Cell Renal Cell Carcinoma via Regulating SLC6A1. Cancer Biol. Ther. 19, 282–291. doi:10.1080/15384047.2017.1394551
Mastroberardino, L., Spindler, B., Pfeiffer, R., Skelly, P. J., Loffing, J., Shoemaker, C. B., et al. (1998). Amino-acid Transport by Heterodimers of 4F2hc/CD98 and Members of a Permease Family. Nature 395, 288–291. doi:10.1038/26246
Mencia, N., Selga, E., Noé, V., and Ciudad, C. J. (2011). Underexpression of miR-224 in Methotrexate Resistant Human Colon Cancer Cells. Biochem. Pharmacol. 82, 1572–1582. doi:10.1016/j.bcp.2011.08.009
Meng, L., Liu, F., Ju, Y., Ding, P., Liu, S., Chang, S., et al. (2018). Tumor Suppressive miR-6775-3p Inhibits ESCC Progression through Forming a Positive Feedback Loop with P53 via MAGE-A Family Proteins. Cell Death Dis. 9, 1057. doi:10.1038/s41419-018-1119-3
Miko, E., Margitai, Z., Czimmerer, Z., Várkonyi, I., Dezső, B., Lányi, Á., et al. (2011). miR-126 Inhibits Proliferation of Small Cell Lung Cancer Cells by Targeting SLC7A5. FEBS Lett. 585, 1191–1196. doi:10.1016/j.febslet.2011.03.039
Milkereit, R., Persaud, A., Vanoaica, L., Guetg, A., Verrey, F., and Rotin, D. (2015). LAPTM4b Recruits the LAT1-4F2hc Leu Transporter to Lysosomes and Promotes mTORC1 Activation. Nat. Commun. 6, 7250. doi:10.1038/ncomms8250
Morris, M. E., and Felmlee, M. A. (2008). Overview of the Proton-Coupled MCT (SLC16A) Family of Transporters: Characterization, Function and Role in the Transport of the Drug of Abuse γ-Hydroxybutyric Acid. AAPS J. 10, 311–321. doi:10.1208/s12248-008-9035-6
Moumné, L., Marie, A. C., and Crouvezier, N. (2022). Oligonucleotide Therapeutics: From Discovery and Development to Patentability. Pharmaceutics 14, 260. doi:10.3390/pharmaceutics14020260
Mueckler, M., and Thorens, B. (2013). The SLC2 (GLUT) Family of Membrane Transporters. Mol. Aspects Med. 34, 121–138. doi:10.1016/j.mam.2012.07.001
O'Brien, J., Hayder, H., Zayed, Y., and Peng, C. (2018). Overview of Microrna Biogenesis, Mechanisms of Actions, and Circulation. Front. Endocrinol. 9, 402. doi:10.3389/fendo.2018.00402
Panda, S., Banerjee, N., and Chatterjee, S. (2020). Solute Carrier Proteins and C-Myc: a Strong Connection in Cancer Progression. Drug Discov. Today 25, 891–900. doi:10.1016/j.drudis.2020.02.007
Papalazarou, V., and Maddocks, O. D. K. (2021). Supply and Demand: Cellular Nutrient Uptake and Exchange in Cancer. Mol. Cell 81, 3731–3748. doi:10.1016/j.molcel.2021.08.026
Park, S. J., Smith, C. P., Wilbur, R. R., Cain, C. P., Kallu, S. R., Valasapalli, S., et al. (2018). An Overview of MCT1 and MCT4 in GBM: Small Molecule Transporters with Large Implications. Am. J. Cancer Res. 8, 1967–1976.
Pasquinelli, A. E., Reinhart, B. J., Slack, F., Martindale, M. Q., Kuroda, M. I., Maller, B., et al. (2000). Conservation of the Sequence and Temporal Expression of Let-7 Heterochronic Regulatory RNA. Nature 408, 86–89. doi:10.1038/35040556
Pavlova, N. N., and Thompson, C. B. (2016). The Emerging Hallmarks of Cancer Metabolism. Cell Metab. 23, 27–47. doi:10.1016/j.cmet.2015.12.006
Payen, V. L., Mina, E., Van Hée, V. F., Porporato, P. E., and Sonveaux, P. (2020). Monocarboxylate Transporters in Cancer. Mol. Metab. 33, 48–66. doi:10.1016/j.molmet.2019.07.006
Pedroza-Torres, A., Romero-Córdoba, S. L., Justo-Garrido, M., Salido-Guadarrama, I., Rodríguez-Bautista, R., Montaño, S., et al. (2019). Micrornas in Tumor Cell Metabolism: Roles and Therapeutic Opportunities. Front. Oncol. 9, 1404. doi:10.3389/fonc.2019.01404
Peng, Y., and Croce, C. M. (2016). The Role of MicroRNAs in Human Cancer. Sig Transduct. Target Ther. 1, 15004. doi:10.1038/sigtrans.2015.4
Pizzagalli, M. D., Bensimon, A., and Superti‐Furga, G. (2021). A Guide to Plasma Membrane Solute Carrier Proteins. FEBS J. 288, 2784–2835. doi:10.1111/febs.15531
Pliszka, M., and Szablewski, L. (2021). Glucose Transporters as a Target for Anticancer Therapy. Cancers (Basel) 13, 4184. doi:10.3390/cancers13164184
Potenza, N., Mosca, N., Mondola, P., Damiano, S., Russo, A., and De Felice, B. (2018). Human miR-26a-5p Regulates the Glutamate Transporter SLC1A1 (EAAT3) Expression. Relevance in Multiple Sclerosis. Biochimica Biophysica Acta (BBA) - Mol. Basis Dis. 1864, 317–323. doi:10.1016/j.bbadis.2017.09.024
Price, N. T., Jackson, V. N., and Halestrap, A. P. (1998). Cloning and Sequencing of Four New Mammalian Monocarboxylate Transporter (MCT) Homologues Confirms the Existence of a Transporter Family with an Ancient Past. Biochem. J. 329 ( Pt 2) (Pt 2), 321–328. doi:10.1042/bj3290321
Pullen, T. J., Da Silva Xavier, G., Kelsey, G., and Rutter, G. A. (2011). miR-29a and miR-29b Contribute to Pancreatic β-Cell-Specific Silencing of Monocarboxylate Transporter 1 (Mct1). Mol. Cell Biol. 31, 3182–3194. doi:10.1128/mcb.01433-10
Qu, W., Ding, S. M., Cao, G., Wang, S. J., Zheng, X. H., and Li, G. H. (2016). miR‐132 Mediates a Metabolic Shift in Prostate Cancer Cells by Targeting Glut1. FEBS open bio 6, 735–741. doi:10.1002/2211-5463.12086
Raal, F. J., Kallend, D., Ray, K. K., Turner, T., Koenig, W., Wright, R. S., et al. (2020). Inclisiran for the Treatment of Heterozygous Familial Hypercholesterolemia. N. Engl. J. Med. 382, 1520–1530. doi:10.1056/nejmoa1913805
Rashid, K., Ahmad, A., Liang, L., Liu, M., Cui, Y., and Liu, T. (2021). Solute Carriers as Potential Oncodrivers or Suppressors: Their Key Functions in Malignant Tumor Formation. Drug Discov. Today 26, 1689–1701. doi:10.1016/j.drudis.2021.03.004
Romero-Cordoba, S. L., Rodriguez-Cuevas, S., Bautista-Pina, V., Maffuz-Aziz, A., D’ippolito, E., Cosentino, G., et al. (2018). Loss of Function of miR-342-3p Results in MCT1 Over-expression and Contributes to Oncogenic Metabolic Reprogramming in Triple Negative Breast Cancer. Sci. Rep. 8, 12252. doi:10.1038/s41598-018-29708-9
Roth, M., Obaidat, A., and Hagenbuch, B. (2012). OATPs, OATs and OCTs: the Organic Anion and Cation Transporters of the SLCO and SLC22A Gene Superfamilies. Br. J. Pharmacol. 165, 1260–1287. doi:10.1111/j.1476-5381.2011.01724.x
Rupaimoole, R., and Slack, F. J. (2017). MicroRNA Therapeutics: towards a New Era for the Management of Cancer and Other Diseases. Nat. Rev. Drug Discov. 16, 203–222. doi:10.1038/nrd.2016.246
Sangani, R., Periyasamy-Thandavan, S., Kolhe, R., Bhattacharyya, M. H., Chutkan, N., Hunter, M., et al. (2015). MicroRNAs-141 and 200a Regulate the SVCT2 Transporter in Bone Marrow Stromal Cells. Mol. Cell. Endocrinol. 410, 19–26. doi:10.1016/j.mce.2015.01.007
Santasusagna, S., Moreno, I., Navarro, A., Muñoz, C., Martinez, F., Hernández, R., et al. (2018). miR-328 Mediates a Metabolic Shift in Colon Cancer Cells by Targeting SLC2A1/GLUT1. Clin. Transl. Oncol. 20, 1161–1167. doi:10.1007/s12094-018-1836-1
Scalise, M., Galluccio, M., Console, L., Pochini, L., and Indiveri, C. (2018). The Human SLC7A5 (LAT1): The Intriguing Histidine/Large Neutral Amino Acid Transporter and its Relevance to Human Health. Front. Chem. 6, 243. doi:10.3389/fchem.2018.00243
Scalise, M., Pochini, L., Galluccio, M., Console, L., and Indiveri, C. (2017). Glutamine Transport and Mitochondrial Metabolism in Cancer Cell Growth. Front. Oncol. 7, 306. doi:10.3389/fonc.2017.00306
Schlessinger, A., Matsson, P., Shima, J. E., Pieper, U., Yee, S. W., Kelly, L., et al. (2010). Comparison of Human Solute Carriers. Protein Sci. 19, 412–428. doi:10.1002/pro.320
Schulte, R. R., and Ho, R. H. (2019). Organic Anion Transporting Polypeptides: Emerging Roles in Cancer Pharmacology. Mol. Pharmacol. 95, 490–506. doi:10.1124/mol.118.114314
Schumann, T., König, J., Henke, C., Willmes, D. M., Bornstein, S. R., Jordan, J., et al. (2020). Solute Carrier Transporters as Potential Targets for the Treatment of Metabolic Disease. Pharmacol. Rev. 72, 343–379. doi:10.1124/pr.118.015735
Schwarz, D. S., Hutvágner, G., Du, T., Xu, Z., Aronin, N., and Zamore, P. D. (2003). Asymmetry in the Assembly of the RNAi Enzyme Complex. Cell 115, 199–208. doi:10.1016/s0092-8674(03)00759-1
Segal, M., and Slack, F. J. (2020). Challenges Identifying Efficacious miRNA Therapeutics for Cancer. Expert Opin. Drug Discov. 15, 987–991. doi:10.1080/17460441.2020.1765770
Setten, R. L., Rossi, J. J., and Han, S.-P. (2019). The Current State and Future Directions of RNAi-Based Therapeutics. Nat. Rev. Drug Discov. 18, 421–446. doi:10.1038/s41573-019-0017-4
Shu, L., Peng, Y., Zhong, L., Feng, X., Qiao, L., and Yi, Y. (2021). CircZNF124 Regulates Cell Proliferation, Leucine Uptake, Migration and Invasion by miR‐199b‐5p/SLC7A5 Pathway in Endometrial Cancer. Immun. Inflamm. Dis. 9, 1291–1305. doi:10.1002/iid3.477
Si, W., Shen, J., Zheng, H., and Fan, W. (2019). The Role and Mechanisms of Action of microRNAs in Cancer Drug Resistance. Clin. Epigenet 11, 25. doi:10.1186/s13148-018-0587-8
Sonveaux, P., Végran, F., Schroeder, T., Wergin, M. C., Verrax, J., Rabbani, Z. N., et al. (2008). Targeting Lactate-Fueled Respiration Selectively Kills Hypoxic Tumor Cells in Mice. J. Clin. Invest. 118, 3930–3942. doi:10.1172/JCI36843
Su, Q., and Wang, H. (2021). Long Non-coding RNA 01559 Mediates the Malignant Phenotypes of Hepatocellular Carcinoma Cells through Targeting miR-511. Clin. Res. Hepatology Gastroenterology 45, 101648. doi:10.1016/j.clinre.2021.101648
Subramanian, V. S., Sabui, S., Marchant, J. S., and Said, H. M. (2019). MicroRNA-103a Regulates Sodium-dependent Vitamin C Transporter-1 Expression in Intestinal Epithelial Cells. J. Nutr. Biochem. 65, 46–53. doi:10.1016/j.jnutbio.2018.12.001
Sun, D., Li, Y.-C., and Zhang, X.-Y. (2021). Lidocaine Promoted Ferroptosis by Targeting miR-382-5p /SLC7A11 Axis in Ovarian and Breast Cancer. Front. Pharmacol. 12, 681223. doi:10.3389/fphar.2021.681223
Sun, R., Ying, Y., Tang, Z., Liu, T., Shi, F., Li, H., et al. (2020). The Emerging Role of the SLCO1B3 Protein in Cancer Resistance. Protein Pept. Lett. 27, 17–29. doi:10.2174/0929866526666190926154248
Takeshita, H., Shiozaki, A., Bai, X.-H., Iitaka, D., Kim, H., Yang, B. B., et al. (2013). XB130, a New Adaptor Protein, Regulates Expression of Tumor Suppressive microRNAs in Cancer Cells. PLoS One 8, e59057. doi:10.1371/journal.pone.0059057
Tu, M.-J., Wright, H. K., Batra, N., and Yu, A.-M. (2021). Expression and of tRNA/pre-miRNA-Based Recombinant Noncoding RNAs. Methods Mol. Biol. 2323, 249–265. doi:10.1007/978-1-0716-1499-0_18
Ubhi, K., Rockenstein, E., Kragh, C., Inglis, C., Spencer, B., Michael, S., et al. (2014). Widespread Micro RNA Dysregulation in Multiple System Atrophy - Disease‐related Alteration in miR‐96. Eur. J. Neurosci. 39, 1026–1041. doi:10.1111/ejn.12444
Ursu, A., Childs-Disney, J. L., Andrews, R. J., O’leary, C. A., Meyer, S. M., Angelbello, A. J., et al. (2020). Design of Small Molecules Targeting RNA Structure from Sequence. Chem. Soc. Rev. 49, 7252–7270. doi:10.1039/d0cs00455c
Van Roosbroeck, K., and Calin, G. A. (2017). Cancer Hallmarks and MicroRNAs: The Therapeutic Connection. Adv. Cancer Res. 135, 119–149. doi:10.1016/bs.acr.2017.06.002
Wang, J., Chen, X., Su, L., Li, P., Cai, Q., Liu, B., et al. (2015). MicroRNA-126 Inhibits Cell Proliferation in Gastric Cancer by Targeting LAT-1. Biomed. Pharmacother. 72, 66–73. doi:10.1016/j.biopha.2015.04.001
Wang, J., Yang, K., Cao, J., and Li, L. (2021). Knockdown of Circular RNA Septin 9 Inhibits the Malignant Progression of Breast Cancer by Reducing the Expression of Solute Carrier Family 1 Member 5 in a microRNA-149-5p-dependent Manner. Bioengineered 12, 10624–10637. doi:10.1080/21655979.2021.2000731
Wang, S.-M., Sun, L.-L., Wu, W.-S., and Yan, D. (2018). MiR-595 Suppresses the Cellular Uptake and Cytotoxic Effects of Methotrexate by TargetingSLC19A1in CEM/C1 Cells. Basic Clin. Pharmacol. Toxicol. 123, 8–13. doi:10.1111/bcpt.12966
Wang, S., Du, H., and Sun, P. (2021). Long Noncoding RNA NEAT1 Contributes to the Tumorigenesis of Colorectal Cancer through Regulating SLC38A1 Expression by Sponging miR-138. Cancer Biotherapy Radiopharm. 36, 793–802. doi:10.1089/cbr.2020.3608
Wang, W. W., Gallo, L., Jadhav, A., Hawkins, R., and Parker, C. G. (2020). The Druggability of Solute Carriers. J. Med. Chem. 63, 3834–3867. doi:10.1021/acs.jmedchem.9b01237
Wang, X., Liu, H., Ni, Y., Shen, P., and Han, X. (2022). Lactate Shuttle: from Substance Exchange to Regulatory Mechanism. Hum. Cell 35, 1–14. doi:10.1007/s13577-021-00622-z
Wang, Y., and Jin, L. (2018). miRNA-145 Is Associated with Spontaneous Hypertension by Targeting SLC7A1. Exp. Ther. Med. 15, 548–552. doi:10.3892/etm.2017.5371
Warburg, O. (1956). On the Origin of Cancer Cells. Science 123, 309–314. doi:10.1126/science.123.3191.309
Wei, Y. B., Melas, P. A., Villaescusa, J. C., Liu, J. J., Xu, N., Christiansen, S. H., et al. (2016). MicroRNA 101b Is Downregulated in the Prefrontal Cortex of a Genetic Model of Depression and Targets the Glutamate Transporter SLC1A1 (EAAT3) In Vitro. Int. J. Neuropsychopharmacol. 19: pyw069. doi:10.1093/ijnp/pyw069
Wei, Z., Liu, X., Cheng, C., Yu, W., and Yi, P. (2020). Metabolism of Amino Acids in Cancer. Front. Cell Dev. Biol. 8, 603837. doi:10.3389/fcell.2020.603837
Wightman, B., Ha, I., and Ruvkun, G. (1993). Posttranscriptional Regulation of the Heterochronic Gene Lin-14 by Lin-4 Mediates Temporal Pattern Formation in C. elegans. Cell 75, 855–862. doi:10.1016/0092-8674(93)90530-4
Wu, K., Xu, T., Song, X., Shen, J., Zheng, S., Zhang, L., et al. (2021). LncRNA SLCO4A1-AS1 Modulates Colon Cancer Stem Cell Properties by Binding to miR-150-3p and Positively Regulating SLCO4A1. Lab. Invest. 101, 908–920. doi:10.1038/s41374-021-00577-7
Wu, Y., Sun, X., Song, B., Qiu, X., and Zhao, J. (2017). MiR-375/SLC7A11 axis Regulates Oral Squamous Cell Carcinoma Proliferation and Invasion. Cancer Med. 6, 1686–1697. doi:10.1002/cam4.1110
Xia, H., Zhang, Z., Yuan, J., and Niu, Q. (2021). The lncRNA PVT1 Promotes Invasive Growth of Lung Adenocarcinoma Cells by Targeting miR-378c to Regulate SLC2A1 Expression. Hum. Cell 34, 201–210. doi:10.1007/s13577-020-00434-7
Xia, Y.-F., Pei, G.-H., Wang, N., Che, Y.-C., Yu, F.-S., Yin, F.-F., et al. (2017). miR-3156-3p Is Downregulated in HPV-Positive Cervical Cancer and Performs as a Tumor-Suppressive miRNA. Virol. J. 14, 20. doi:10.1186/s12985-017-0695-7
Xiao, D., Zhou, T., Fu, Y., Wang, R., Zhang, H., Li, M., et al. (2018). MicroRNA-17 Impairs Glucose Metabolism in Insulin-Resistant Skeletal Muscle via Repressing Glucose Transporter 4 Expression. Eur. J. Pharmacol. 838, 170–176. doi:10.1016/j.ejphar.2018.08.036
Xiao, W., Wang, X., Wang, T., and Xing, J. (2019). MiR-223-3p Promotes Cell Proliferation and Metastasis by Downregulating SLC4A4 in Clear Cell Renal Cell Carcinoma. Aging 11, 615–633. doi:10.18632/aging.101763
Xu, H., Wang, X., Wu, J., Ji, H., Chen, Z., Guo, H., et al. (2020). Long Non-coding RNA LINC01094 Promotes the Development of Clear Cell Renal Cell Carcinoma by Upregulating SLC2A3 via MicroRNA-184. Front. Genet. 11, 562967. doi:10.3389/fgene.2020.562967
Yamasaki, T., Seki, N., Yoshino, H., Itesako, T., Yamada, Y., Tatarano, S., et al. (2013). Tumor-suppressivemicroRNA-1291directly Regulates Glucose Transporter 1 in Renal Cell Carcinoma. Cancer Sci. 104, 1411–1419. doi:10.1111/cas.12240
Yan, S.-T., Li, C.-L., Tian, H., Li, J., Pei, Y., Liu, Y., et al. (2014). MiR-199a Is Overexpressed in Plasma of Type 2 Diabetes Patients Which Contributes to Type 2 Diabetes by Targeting GLUT4. Mol. Cell Biochem. 397, 45–51. doi:10.1007/s11010-014-2170-8
Yang, L., Venneti, S., and Nagrath, D. (2017). Glutaminolysis: A Hallmark of Cancer Metabolism. Annu. Rev. Biomed. Eng. 19, 163–194. doi:10.1146/annurev-bioeng-071516-044546
Yang, X., Tao, Z., Zhu, Z., Liao, H., Zhao, Y., and Fan, H. (2016). MicroRNA-593-3p Regulates Insulin-Promoted Glucose Consumption by Targeting Slc38a1 and CLIP3. J. Mol. Endocrinol. 57, 211–222. doi:10.1530/jme-16-0090
Yang, Y., Tai, W., Lu, N., Li, T., Liu, Y., Wu, W., et al. (2020). lncRNA ZFAS1 Promotes Lung Fibroblast-To-Myofibroblast Transition and Ferroptosis via Functioning as a ceRNA through miR-150-5p/SLC38A1 axis. Aging 12, 9085–9102. doi:10.18632/aging.103176
Yarmishyn, A. A., Ishola, A. A., Chen, C. Y., Verusingam, N. D., Rengganaten, V., Mustapha, H. A., et al. (2022). Circular RNAs Modulate Cancer Hallmark and Molecular Pathways to Support Cancer Progression and Metastasis. Cancers (Basel) 14, 862. doi:10.3390/cancers14040862
Yasukawa, K., Kinoshita, D., Yaku, K., Nakagawa, T., and Koshiba, T. (2020). The microRNAs miR-302b and miR-372 Regulate Mitochondrial Metabolism via the SLC25A12 Transporter, Which Controls MAVS-Mediated Antiviral Innate Immunity. J. Biol. Chem. 295, 444–457. doi:10.1074/jbc.ra119.010511
Young, J. D., Yao, S. Y., Baldwin, J. M., Cass, C. E., and Baldwin, S. A. (2013). The human concentrative and equilibrative nucleoside transporter families, SLC28 and SLC29. Mol. Aspects Med. 34, 529–547. doi:10.1016/j.mam.2012.05.007
Yi, W., Tu, M.-J., Liu, Z., Zhang, C., Batra, N., Yu, A.-X., et al. (2020). Bioengineered miR-328-3p Modulates GLUT1-Mediated Glucose Uptake and Metabolism to Exert Synergistic Antiproliferative Effects with Chemotherapeutics. Acta Pharm. Sin. B 10, 159–170. doi:10.1016/j.apsb.2019.11.001
Yu, A.-M., Choi, Y. H., and Tu, M.-J. (2020). RNA Drugs and RNA Targets for Small Molecules: Principles, Progress, and Challenges. Pharmacol. Rev. 72, 862–898. doi:10.1124/pr.120.019554
Yu, A.-M., and Pan, Y.-Z. (2012). Noncoding microRNAs: Small RNAs Play a Big Role in Regulation of ADME? Acta Pharm. Sin. B 2, 93–101. doi:10.1016/j.apsb.2012.02.011
Yu, A.-M., Tian, Y., Tu, M.-J., Ho, P. Y., and Jilek, J. L. (2016). MicroRNA Pharmacoepigenetics: Posttranscriptional Regulation Mechanisms behind Variable Drug Disposition and Strategy to Develop More Effective Therapy. Drug Metabolism Dispos. 44, 308–319. doi:10.1124/dmd.115.067470
Yu, A.-M., and Tu, M.-J. (2022). Deliver the Promise: RNAs as a New Class of Molecular Entities for Therapy and Vaccination. Pharmacol. Ther. 230, 107967. doi:10.1016/j.pharmthera.2021.107967
Yu, D., Tolleson, W. H., Knox, B., Jin, Y., Guo, L., Guo, Y., et al. (2015). Modulation of ALDH5A1 and SLC22A7 by microRNA Hsa-miR-29a-3p in Human Liver Cells. Biochem. Pharmacol. 98, 671–680. doi:10.1016/j.bcp.2015.09.020
Yu, J., Chen, X., Li, J., and Wang, F. (2021). CircRUNX1 Functions as an Oncogene in Colorectal Cancer by Regulating circRUNX1/miR-485-5p/SLC38A1 axis. Eur. J. Clin. Invest. 51, e13540. doi:10.1111/eci.13540
Yu, S., Wu, Y., Li, C., Qu, Z., Lou, G., Guo, X., et al. (2020). Comprehensive Analysis of the SLC16A Gene Family in Pancreatic Cancer via Integrated Bioinformatics. Sci. Rep. 10, 7315. doi:10.1038/s41598-020-64356-y
Zhang, B., Liu, T., Wu, T., Wang, Z., Rao, Z., and Gao, J. (2015). microRNA-137 Functions as a Tumor Suppressor in Human Non-small Cell Lung Cancer by Targeting SLC22A18. Int. J. Biol. Macromol. 74, 111–118. doi:10.1016/j.ijbiomac.2014.12.002
Zhang, J.-X., Xu, Y., Gao, Y., Chen, C., Zheng, Z.-S., Yun, M., et al. (2017). Decreased Expression of miR-939 Contributes to Chemoresistance and Metastasis of Gastric Cancer via Dysregulation of SLC34A2 and Raf/MEK/ERK Pathway. Mol. Cancer 16, 18. doi:10.1186/s12943-017-0586-y
Zhang, M. M., Bahal, R., Rasmussen, T. P., Manautou, J. E., and Zhong, X.-B. (2021). The Growth of siRNA-Based Therapeutics: Updated Clinical Studies. Biochem. Pharmacol. 189, 114432. doi:10.1016/j.bcp.2021.114432
Zhang, T., Li, M., Lu, H., and Peng, T. (2021). Up-Regulation of circEIF6 Contributes to Pancreatic Cancer Development through Targeting miR-557/SLC7A11/PI3K/AKT Signaling. Cancer Manag. Res. 13, 247–258. doi:10.2147/cmar.s280307
Zhang, X., Tan, P., Zhuang, Y., and Du, L. (2020). hsa_circRNA_001587 Upregulates SLC4A4 Expression to Inhibit Migration, Invasion, and Angiogenesis of Pancreatic Cancer Cells via Binding to microRNA-223. Am. J. Physiology-Gastrointestinal Liver Physiology 319, G703–G717. doi:10.1152/ajpgi.00118.2020
Zhang, Y., Zhang, Y., Sun, K., Meng, Z., and Chen, L. (2019). The SLC Transporter in Nutrient and Metabolic Sensing, Regulation, and Drug Development. J. Mol. Cell Biol. 11, 1–13. doi:10.1093/jmcb/mjy052
Zheng, T., Chen, W., Wang, X., Cai, W., Wu, F., and Lin, C. (2021). Circular RNA Circ-Fam158a Promotes Retinoblastoma Progression by Regulating miR-138-5p/SLC7A5 axis. Exp. Eye Res. 211, 108650. doi:10.1016/j.exer.2021.108650
Zhou, F., Zhu, L., Wang, K., and Murray, M. (2017). Recent Advance in the Pharmacogenomics of Human Solute Carrier Transporters (SLCs) in Drug Disposition. Adv. Drug Deliv. Rev. 116, 21–36. doi:10.1016/j.addr.2016.06.004
Zhou, S., Zhang, D., Guo, J., Chen, Z., Chen, Y., and Zhang, J. (2020). Long Non‐coding RNA NORAD Functions as a microRNA‐204‐5p Sponge to Repress the Progression of Parkinson's Disease In Vitro by Increasing the Solute Carrier Family 5 Member 3 Expression. IUBMB Life 72, 2045–2055. doi:10.1002/iub.2344
Zhou, T., Meng, X., Che, H., Shen, N., Xiao, D., Song, X., et al. (2016). Regulation of Insulin Resistance by Multiple MiRNAs via Targeting the GLUT4 Signalling Pathway. Cell Physiol. Biochem. 38, 2063–2078. doi:10.1159/000445565
Zhu, J.-H., De Mello, R. A., Yan, Q.-L., Wang, J.-W., Chen, Y., Ye, Q.-H., et al. (2020). MiR-139-5p/SLC7A11 Inhibits the Proliferation, Invasion and Metastasis of Pancreatic Carcinoma via PI3K/Akt Signaling Pathway. Biochimica Biophysica Acta (BBA) - Mol. Basis Dis. 1866, 165747. doi:10.1016/j.bbadis.2020.165747
Keywords: microRNA, nutrient, xenobiotic, therapy, cancer, solute carrier, regulation, therapy
Citation: Yi C and Yu A-M (2022) MicroRNAs in the Regulation of Solute Carrier Proteins Behind Xenobiotic and Nutrient Transport in Cells. Front. Mol. Biosci. 9:893846. doi: 10.3389/fmolb.2022.893846
Received: 10 March 2022; Accepted: 02 May 2022;
Published: 09 June 2022.
Edited by:
Luca Mollica, University of Milan, ItalyReviewed by:
Francesca Anna Cupaioli, National Research Council of Italy, ItalyShushi Nagamori, Jikei University School of Medicine, Japan
Copyright © 2022 Yi and Yu. This is an open-access article distributed under the terms of the Creative Commons Attribution License (CC BY). The use, distribution or reproduction in other forums is permitted, provided the original author(s) and the copyright owner(s) are credited and that the original publication in this journal is cited, in accordance with accepted academic practice. No use, distribution or reproduction is permitted which does not comply with these terms.
*Correspondence: Ai-Ming Yu, aimyu@ucdavis.edu