The Neuroprotective Beta Amyloid Hexapeptide Core Reverses Deficits in Synaptic Plasticity in the 5xFAD APP/PS1 Mouse Model
- Department of Cell & Molecular Biology, John A. Burns School of Medicine, University of Hawai‘i at Mānoa, Honolulu, HI, United States
Alzheimer’s disease (AD) is the most common cause of dementia in the aging population. Evidence implicates elevated soluble oligomeric Aβ as one of the primary triggers during the prodromic phase leading to AD, effected largely via hyperphosphorylation of the microtubule-associated protein tau. At low, physiological levels (pM-nM), however, oligomeric Aβ has been found to regulate synaptic plasticity as a neuromodulator. Through mutational analysis, we found a core hexapeptide sequence within the N-terminal domain of Aβ (N-Aβcore) accounting for its physiological activity, and subsequently found that the N-Aβcore peptide is neuroprotective. Here, we characterized the neuroprotective potential of the N-Aβcore against dysfunction of synaptic plasticity assessed in ex vivo hippocampal slices from 5xFAD APP/PS1 mice, specifically hippocampal long-term potentiation (LTP) and long-term depression (LTD). The N-Aβcore was shown to reverse impairment in synaptic plasticity in hippocampal slices from 5xFAD APP/PS1 model mice, both for LTP and LTD. The reversal by the N-Aβcore correlated with alleviation of downregulation of hippocampal AMPA-type glutamate receptors in preparations from 5xFAD mice. The action of the N-Aβcore depended upon a critical di-histidine sequence and involved the phosphoinositide-3 (PI3) kinase pathway via mTOR (mammalian target of rapamycin). Together, the present findings indicate that the non-toxic N-Aβcore hexapeptide is not only neuroprotective at the cellular level but is able to reverse synaptic dysfunction in AD-like models, specifically alterations in synaptic plasticity.
Introduction
Alzheimer’s disease (AD) is clinically characterized by impairments in cognitive memory and function. Loss of critical pre- and post-synaptic markers have been reported for postmortem AD brain tissue (Reddy et al., 2005; Scheff et al., 2006), suggesting that AD-related cognitive impairments are based, in large part, on synaptic dysfunction and loss. Additionally, accumulating evidence shows a strong link between excess soluble oligomeric amyloid-β (Aβ) and synaptic dysfunction in AD (Walsh et al., 2002; Glabe, 2005; Shankar and Walsh, 2009). Cognitive decline and synaptic plasticity deficits are reported to occur prior to the accumulation of Aβ plaques and tau neurofibrillary tangles in the prodromic phase leading to AD (Oddo et al., 2003), supporting the idea that synaptic dysfunction and mild cognitive impairment are early events driven by soluble oligomeric Aβ rising to abnormally high levels years prior to AD diagnosis.
Synaptic dysfunction and eventual degeneration lead to abnormal synaptic transmission and impaired long-term potentiation (LTP) and/or long-term depression (LTD), which are important in synaptic plasticity and learning and memory. Pathological levels (high nM to μM) of Aβ have been shown to inhibit LTP-induction (Cullen et al., 1997; Chapman et al., 1999; Walsh et al., 2002) and enhance LTD (Li et al., 2009; Chen et al., 2013) in the hippocampus. On the other hand, low physiological levels (pM) of Aβ was found to enhance LTP and memory, indicating a hormetic effect of Aβ on synaptic plasticity (Puzzo et al., 2008, 2012; Lawrence et al., 2014; Gulisano et al., 2019).
Dysregulation of synaptic plasticity in AD pathogenesis involves altered regulation of NMDA-type and AMPA-type glutamate receptors. In addition to mediating Aβ-induced excitotoxicity, NMDA receptors can be depressed by Aβ at high concentrations (Snyder et al., 2005), inducing LTD (Hsieh et al., 2006; D’Amelio et al., 2011) as a consequence of subsequent downstream AMPA receptor internalization (Hsieh et al., 2006; D’Amelio et al., 2011) and dendritic spine loss (D’Amelio et al., 2011).
We have shown that at low concentration (pM-nM) the N-terminal Aβ fragment comprising amino acids 1–15/16 of the Aβ sequence, an endogenous peptide cleaved from Aβ via α-secretase (Portelius et al., 2011), is more effective as a neuromodulator than full-length Aβ1–42, stimulating receptor-linked increases in neuronal Ca2+, enhancing synaptic plasticity and enhancing contextual fear memory (Lawrence et al., 2014). The Aβ1–16 peptide sequence corresponds to the C-terminal 16 amino acid sequence in soluble amyloid precursor protein-α (sAPP-α), referred to as the CTα16, which has also been shown to enhance synaptic plasticity (Morrisey et al., 2019). An essential core sequence comprising amino acids 10–15 of Aβ (N-Aβcore) was identified as the active region of the N-terminal Aβ fragment and was further shown to protect against Aβ-induced neuronal toxicity (Forest et al., 2018). Here, we aimed to better understand the neuroprotective mechanism of the N-Aβcore on synaptic plasticity. We investigated whether the N-Aβcore could rescue LTP and LTD dysfunction resulting from prolonged, elevated levels of Aβ in an APP/PS1 transgenic mouse model harboring mutations found in familial Alzheimer’s disease (FAD), while assessing the impact on AMPA-type glutamate receptor expression in reference to the neuroprotective action of the N-Aβcore in Aβ-synaptotoxicity (Forest et al., 2020).
Materials and Methods
Transgenic Mice and Cannulation Surgery
All animal handling, surgery, use and euthanasia were performed under approved IACUC protocols (11-1219-6/16-2282-2), compliant with NIH and Society for Neuroscience guidelines for use of vertebrate animals in neuroscience research. The human APP/PSEN1 mouse line, 5xFAD (Tg6799), on the B6.SJL background (B6SJL-Tg (APPSwFlLOn, PSEN1∗M146L∗L286V) 6799 Vas/Mmjax; obtained from JAX stock #006554, MMRRC034840 hemizygous) was used as a well characterized model for human Aβ-based FAD pathology and neurodegeneration (Oakley et al., 2006), with noted limitations in regard to its application in a physiological context. As the transgenic mice are hemizygous, age-matched B6.SJL background mice (MMRRC034840 Non-carrier) were used as controls. Aged adult mice at 7- to 8-months of age of both sexes (weight range: 28–35 g), obtained from established in-house colonies and housed in ventilated enrichment cages in the John A. Burns School of Medicine AAALAC-accredited Vivarium with ad libitum access to food and water, were used at roughly equal numbers, this age range selected for displaying pronounced LTP deficits in the transgenic line. For comparison of B6.SJL mice for baseline treatment, adult mice at 4–5 months of age were used in one set of experiments. Inclusion/exclusion criteria were based on animal health.
For bilateral cannulation and injection, the following protocol was employed (as per Forest et al., 2018). Cannulation into the dorsal aspects of both hippocampi of the 5xFAD mice at 7- to 8-months of age of both sexes was performed under full anesthesia (general: 1.2% Avertin; local at site: lidocaine) using stereotaxis (coordinates: −1.5 mm anteroposterior; ±1 mm lateral; −2 mm depth). After the brief surgical procedure and recovery (full righting reflex), mice were subsequently housed in sound-isolated, ventilated hotels prior to peptide injection one week later. On the day of microinjection (morning), sterile saline or 500 nM N-Aβcore peptide was administered bilaterally through the cannulae in the 5xFAD mice via microinjectors over 30s (0.5μL/side) and the mice were returned to their cages in the mouse hotel. Hippocampi were collected from euthanized mice 24 h after the bilateral microinjection of the saline or peptide, and lysates extracted from the hippocampi were prepared for immunoblot analysis (30 μg each). Euthanasia was performed under approved IACUC protocols (11-1219-6/16-2282-2). This study was not preregistered and followed ARRIVE guidelines.
Aβ Peptides
Solutions of Aβ1–42 (American Peptide; Anaspec) were prepared from aqueous stock solutions, followed by bath sonication. This preparation of full-length Aβ (Aβ1–42) was previously shown to exist predominantly in the oligomeric state (see Lawrence et al., 2014). The N-Aβcore peptide, YEVHHQ, and the substituted peptide, SEVAAQ, previously shown to be inactive (Forest et al., 2018), and here used as a control, were prepared from aqueous stock solutions of peptides synthesized and isolated at >98% purity (Peptide 2.0). Concentrations of the Aβ peptides used were based on previous studies of synaptic plasticity (Lawrence et al., 2014).
Extracellular Field Potential Recordings in Hippocampal Slices
Hippocampal slices were prepared from aged adult (4- or 7- to 8- month-old) B6.SJL (control) or 5xFAD (Tg6799) mice (as per Lawrence et al., 2014). Cervical dislocation and decapitation were performed under approved IACUC protocols (11-1219-6/16-2282-2), compliant with NIH and Society for Neuroscience guidelines for use of vertebrate animals in neuroscience research. Brains were removed into ice-cold artificial cerebral spinal fluid (aCSF) consisting of 130 mM NaCl, 3.5 mM KCl, 10 mM glucose, 1.25 mM NaH2PO4, 2.0 mM CaCl2, 1.5 mM MgSO4, and 24 mM NaHCO3, bubbled in 95% O2/5% CO2. Transverse brain slices of 400μm were obtained using a Leica vibrating microtome (Leica, VT1200S) and quickly transferred to fresh ice-cold aCSF for hippocampi isolation. Extracted hippocampi slices were incubated in bubbled aCSF in a holding chamber for 30 min at room temperature (23°C) after which the holding chamber was transferred to a 32°C water bath for 1 h. The chamber was then removed from the water bath and placed at room temperature for another 1 h prior to recording. The slices were subsequently transferred to a recording chamber and perfused at 3 mL/min with aCSF (bubbled with 95% O2/5% CO2) at 32°C. The Schaffer collateral fibers were stimulated at a frequency 0.1 Hz using a bipolar stimulating electrode and CA1 field excitatory postsynaptic potentials (fEPSPs) were recorded with a glass electrode filled with 3M NaCl (resistance 1–1.5 MΩ). Basal synaptic transmission was assessed by comparing stimulus strength against fEPSP slope to generate input/output (I/O) curves. A minimum of 20 min baseline stimulation was then performed, recording every minute. The baseline and stimulus current were adjusted during this period so that fEPSP stabilized at 30–40% of maximum amplitude.
Long-term potentiation was induced by a 3-theta-burst stimulation (TBS) protocol, where each burst consisted of four pulses at 100 Hz with a 200-ms interburst interval. LTD was induced using a low frequency stimulation (LFS) protocol, consisting of a 1 Hz single pulse stimulus (900 pulses for 15 min). TBS and LFS were administered after a 20-min baseline recording period for aCSF alone or a 35-min baseline recording period in aCSF (15 min) followed by inclusion of N-Aβcore in the absence or presence of tested reagents (20 min). For the latter, TBS and LFS were administered in the presence of N-Aβcore in the absence or presence of tested reagents. The phosphoinositide-3 (PI3) kinase inhibitor LY294002 (Sigma, # 440202) and the mTOR inhibitor rapamycin (Sigma, #553210) were used at effective concentrations based on prior studies (Hou and Klann, 2004).
Immunoblot Analysis
Hippocampi Injected With N-Aβcore
Hippocampi removed from euthanized 8-month-old 5xFAD mice previously bilaterally injected with saline or N-Aβcore were homogenized with 250 μL of Pierce IP Lysis Buffer (Thermo Fisher Scientific, # 87788, lot# MJ162614) with 1× Halt Protease and Phosphatase Inhibitor Cocktail (Thermo Fisher Scientific, # 78441, lot# SF248390). The homogenates were centrifuged at 18,000 × g for 20 min at 4°C and the supernatant was collected. The total amount of protein was quantified by a PierceTM BCA Protein Assay Kit (Thermo Fisher Scientific, # 23225).
For each condition, gel sample buffer (4×; Thermo Fisher Scientific, # B0007, lot # 1920132) and reducing agent (10×; Thermo Fisher Scientific, # B0009, lot # 1901009) were added to diluted SDS-solubilized protein samples for a final protein concentration of 2 μg/μL. The samples were boiled at 95°C for 10 min, immediately cooled on ice and then centrifuged. Equal amounts of protein were subjected to electrophoretic separation on a 4–20% Tris-Glycine polyacrylamide gel (Thermo Fisher Scientific, #XP04200), transferred to Nitrocellulose membrane (LI-COR, # 92631092) via the iBlot2 semidry system (Thermo Fisher Scientific). Recovered blots were incubated in primary antibody (see below) overnight at 4°C. The transfer blots were washed 3× (10 min each wash) in 0.1% Tween-20 in Tris-buffered saline and incubated in the appropriate IR-dye-conjugated secondary antibody (LI-COR Biosciences) for 1 h. An Odyssey IR imaging system (LI-COR Biosciences, Lincoln, NE) was used for signal detection. Analysis was performed via Image Studio v5.2.5 software (LI-COR Biosciences).
The following primary antibodies were used for detection and normalization, respectively: anti-pAMPAR1 rabbit monoclonal antibody (pS831-GluA1; Cell Signaling Technology; RRID:AB_2799873) and anti-AMPAR1 rabbit monoclonal antibody (GluA1; Cell Signaling Technology; RRID:AB_2732897), both at 1:1000 and anti-beta actin mouse monoclonal antibody (Sigma-Aldrich; RRID:AB_476697) at 1:10,000 dilution.
Cultured Hippocampal Neurons
Hippocampal neuron cultures were prepared as described (Cheng and Yakel, 2015) from neonatal mouse pups (0–2 days old; 1 litter (6–10 mice)/preparation) from female breeders housed in the John A. Burns School of Medicine AAALAC-accredited Vivarium of either gender (equivalent numbers) obtained from established colonies of wild-type B6.SJL (background) mice. All animal procedures (handling; euthanasia) followed an IACUC-approved protocol (16-2282-2), compliant with NIH and Society for Neuroscience guidelines for use of vertebrate animals in neuroscience research. Following rapid decapitation, brains were removed from the mice into ice-cold Neurobasal A medium (NB) containing B-27 supplement, 5% fetal bovine serum (FBS) and Gentamicin. Hippocampi were then dissected under a stereomicroscope (Olympus SZ61). The isolated hippocampi were treated with papain (Worthington, LS003126, Lot # 35N16202) in Hanks buffer with 10 mM cysteine at 37°C for 15 min. The partially digested tissue was washed by centrifugation in NB plus FBS. The cells were dissociated using sequential trituration (polished Pasteur pipettes of decreasing diameter) and collected by low-speed centrifugation. The dissociated cells were pre-plated in standard tissue culture dishes to remove adherent non-neuronal cells (glia; fibroblasts) for 10–15 min. The neuron-enriched preparation was diluted to 1 × 105 cells/mL and then plated into poly-D-lysine-coated 24-well dishes in NB plus serum and Gentamicin. The cultures were washed with Neurobasal A medium containing B27 and Gentamicin to remove the serum and then cultured in this media for 7–10 days prior to treatment with Aβ, N-Aβcore or the combination for an additional 7 days.
qPCR
RNA was extracted from treated cultured hippocampal neurons using the PureLink® RNA Mini Kit (Ambion, Life Technologies, #12183025) as per the manufacturer’s protocol. Genomic DNA contamination was eliminated from the RNA preparation by digesting with RNase-free DNase (Qiagen, #79254). The iScript cDNA Synthesis Kit (Bio-Rad) was used to synthesize cDNA. The expression levels of various genes were then determined using SYBR green via qPCR (Bio-Rad iCycler iQTM Multicolor Real-Time PCR Detection System) using the primers listed in Table 1. Cycling conditions were as follows: 95°C for 15 min, followed by 40 cycles of 94°C for 15 s and 60°C for 60 s and the fold-changes in the variously treated samples compared to untreated (vehicle control) samples were calculated after normalizing to GAPDH gene expression.
Data and Statistical Analysis
Treatment and units were randomized as to order for all assays and experiments. Biological replicates were based on independent samples (n). For extracellular recording, each biological replicate was one mouse hippocampal slice (only one slice from one mouse hippocampus per condition), unless otherwise noted. All experiments were repeated at least three times, unless otherwise noted. After testing for normality, multiple comparisons of the data were made using one-way ANOVA with Bonferroni or Tukey’s post hoc tests, as indicated. Paired comparison was made using Student’s t-tests, or as appropriate, Chi-square test. P-values < 0.05 were considered the minimum for significance (as rejection of the null hypothesis). Unless otherwise noted, data were analyzed and graphed with GraphPad Prism 5 (GraphPad v5.0b; RRID:SCR_002798) using the appropriate statistical tests.
Results
The N-Aβcore Reversed LTP Deficits Induced by Pathological Levels of Full-Length Aβ
We have previously shown that the N-terminal Aβ fragment (Aβ1–15/16) enhances synaptic plasticity and contextual fear memory while protecting against Aβ-linked synaptic impairment (Lawrence et al., 2014). Considering the evidence that the N-Aβcore hexapeptide, YEVHHQ, accounts for the neuromodulatory activity of the N-terminal Aβ fragment, we assessed whether the N-Aβcore is capable of reversing Aβ-linked synaptic dysfunction in an ex vivo model. We utilized hippocampal slices from a mutant APP/PS1 transgenic AD-like endophenotype mouse model (5xFAD) and their wild-type counterparts (B6.SJL) to examine synaptic transmission. Basal synaptic transmission at the Schaffer collateral-CA1 synapses represented by input-output curves shows that the fEPSP slopes versus stimulus strength for both the 5xFAD and B6.SJL control mice were comparable (Figure 1A), ruling out any issues with regard to impact of the Aβ fragment peptides on baseline synaptic strength. Treatment with 500 nM N-Aβcore during baseline recordings induced increases in baseline synaptic transmissions for both 5xFAD and B6.SJL, but was only significant in the B6.SJL slices (Figure 1B; average increase relative to untreated controls: 110% ± 2% SD), similar to that observed for the N-terminal Aβ fragment over the same time period (Lawrence et al., 2014), where the increased fEPSPs were found to extend through the high-frequency stimulation and post-tetanic potentiation (see Figure 1C).
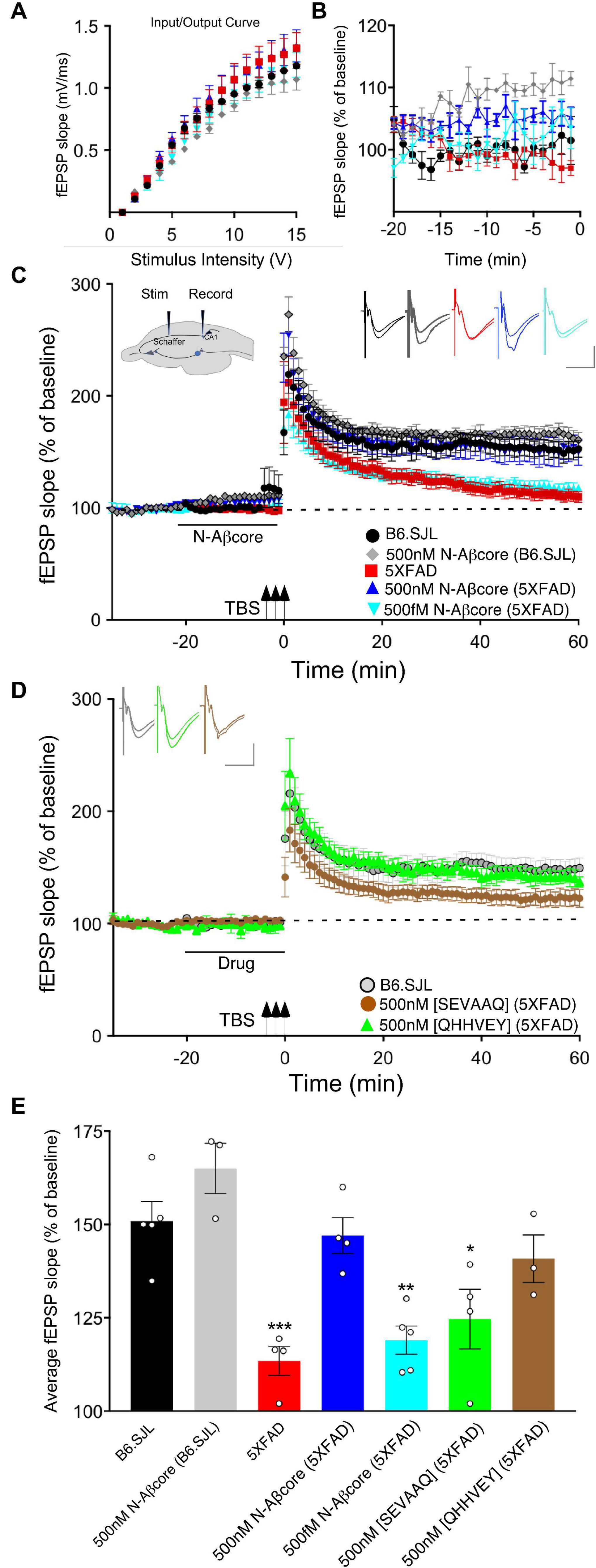
Figure 1. The N-Aβcore reverses Aβ-induced synaptic impairment in APP/PSEN1 mutant mouse hippocampal slices. Input/output curves (A) plotting average fEPSP slopes versus stimulus strength before treatment and baseline recordings compared to panel (B) during the drug pretreatment period versus aCSF perfusion alone prior to stimulation for: control aCSF in B6.SJL slices (black, n = 5), 5xFAD slices (red, n = 4), 500 nM N-Aβcore in B6.SJL slices (gray, n = 3) and 5xFAD slices (blue, n = 4), plus 500 fM. N-Aβcore in 5xFAD slices (cyan, n = 5). No statistically significant differences in the input/output curves (A) were found (p > 0.05, one-way repeated measures ANOVA). Averaged (last 10 min**) baseline data (B) were analyzed by one-way ANOVA (F(4,16) = 7.53, p = 0.0013). Bonferroni post hoc tests revealed significant enhancement of baseline fEPSPs by 500 nM N-Aβcore on B6.SJL slices compared to aCSF-perfused B6.SJL slices (p = 0.006), aCSF perfused 5xFAD slices (p = 0.002), and by 500 fM N-Aβcore on 5xFAD slices (p = 0.038). (C) TBS-induced LTP for B6.SJL slices perfused with control aCSF (black, N = 5) or 500 nM N-Aβcore (gray, N = 3) and 5xFAD slices with aCSF (red, N = 4), 500 nM N-Aβcore (blue, N = 4) and 500 fM N-Aβcore (cyan, N = 5). Inset in panel (C), diagram of hippocampal slice stimulation of the Schaffer collaterals (Stim) and recording fEPSPs in CA1 (Record). (D) TBS-induced LTP for 5xFAD slices treated with 500 nM [SEVAAQ] (green, n = 5) or 500 nM [QHHVEY] (brown, n = 3) substituted N-Aβcore peptides, and, as a reference, B6.SJL perfused with control aCSF [gray circles, n = 5, data from panel (C)]. (E) Quantification of average fEPSP slope values for 50–60 min post TBS. All groups shown in panels (C,D) were analyzed by one-way ANOVA (F(6,21) = 11.33, p < 0.0001). Bonferroni post hoc tests: differences found for 5xFAD slices (p = 0.0012), 500 fM N-Aβcore in 5xFAD slices (p = 0.0036), and 500 nM [SEVAAQ] in 5xFAD slices (p = 0.041) when compared to aCSF-perfused (control) B6.SJL slices. Application of 500 nM N-Aβcore in 5xFAD slices reversed the LTP deficit in the 5xFAD slices (p < 0.0005). Horizontal bars in panels (C,D) indicate the period (20 min) of drug delivery for the color-coded conditions as indicated. Arrows indicate timing of TBS in panels (C,D). Color-coded insets showing examples of fEPSPs (baseline vs. LTP). All data are means ± SEM. N values represent independent experiments (1 slice/mouse). Inset calibration: horizontal, 10 ms; vertical, 0.4 mV. *p < 0.05; **p < 0.005, ***p < 0.0005.
To assess sustained changes in synaptic plasticity, we used a 3×-TBS stimulation protocol at the Schaffer collaterals to measure LTP in the CA1 region (see cross-sectional diagram of the hippocampus in Figure 1A, inset). LTP showed a trend toward enhancement for the N-Aβcore-treated B6.SJL slices as compared to untreated slice, though it was not significant (Figures 1C,E). Consistent with previous findings (Crouzin et al., 2013), LTP in the 5xFAD slices was substantially reduced compared to that observed for slices from B6.SJL (Figures 1C,E; 24.2% of control), dropping to near baseline at 60 min post-TBS. By contrast, prestimulation treatment with 500 nM N-Aβcore restored LTP in the 5xFAD slices to the level seen for the wild-type slices (Figures 1C,E; 107% ± 44% SD of control). These findings demonstrate that the N-Aβcore can reverse LTP deficits induced from prolonged exposure to pathological levels of Aβ, while modestly enhancing basal synaptic transmission.
The N-Aβcore Reversed Full-Length Aβ-Linked Downregulation of Hippocampal AMPA-Type Glutamate Receptors
Regulation of synaptic expression of AMPA-type glutamate receptors (AMPARs) has been shown to underlie LTP (Makino and Malinow, 2009; Huganir and Nicoll, 2013). As downregulation of AMPARs is linked to the impairment in hippocampal LTP in APP/PS1 mice (Hsieh et al., 2006), the impact in vitro and in vivo of the N-Aβcore on the regulation of hippocampal AMPARs was assessed.
Utilizing hippocampal neuronal cultures derived from the background wild-type mice, B6.SJL, and treated with exogenous full-length Aβ (Aβ42) for 7 days, Aβ42 was shown to downregulate AMPAR1 (GluA1) transcript expression assessed via qPCR in this in vitro Aβ toxicity model as compared to untreated control cultures (Figure 2A), consistent with previous findings. There was no significant impact of the N-Aβcore compared to control, while there was a modest trend for the N-Aβcore on the downregulation by Aβ42. There was no impact of any condition on AMPAR2 (GluA2) transcript expression.
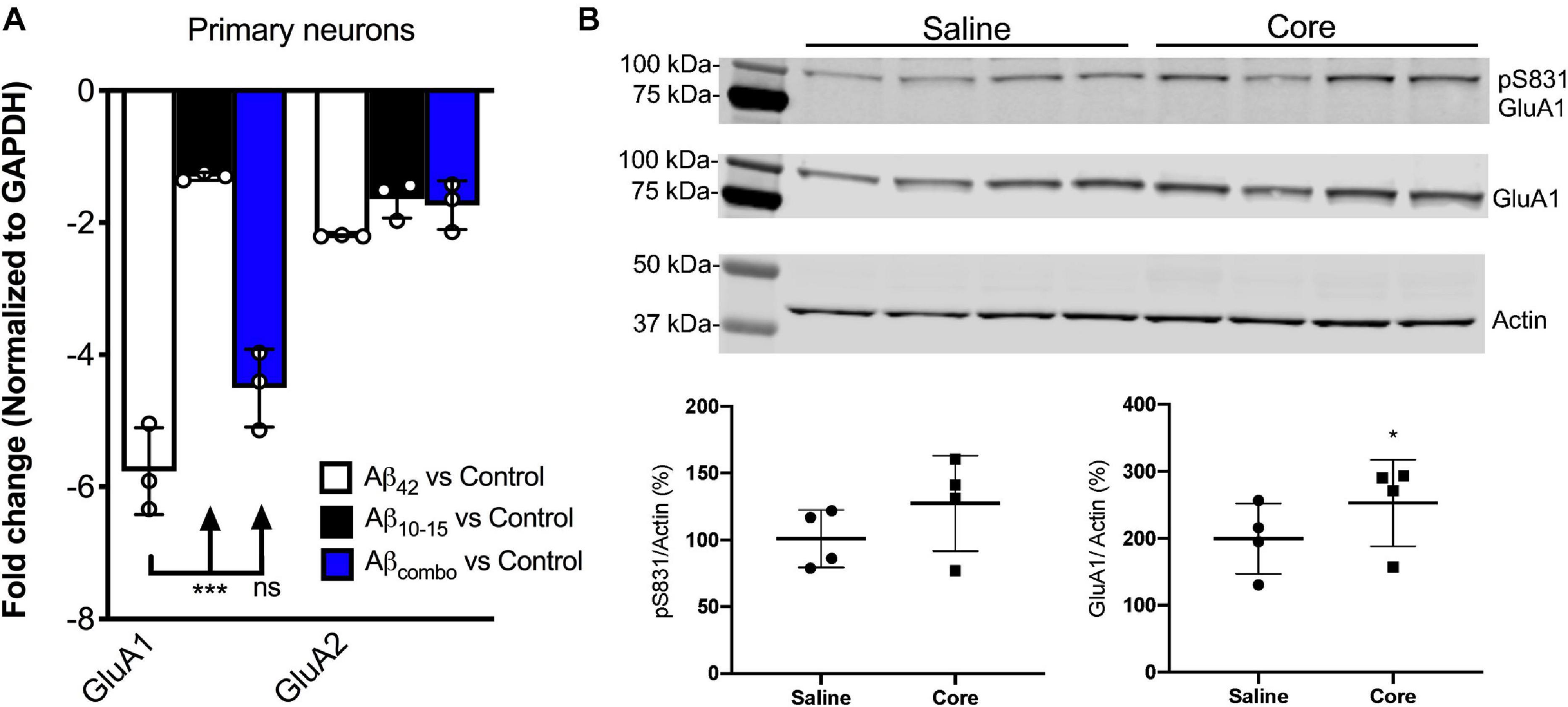
Figure 2. N-AβCore normalizes AMPAR1 expression in an in vitro Aβ neurotoxicity model of primary hippocampal neurons and in vivo in hippocampi of 5xFAD APP/PS1 mice. (A) Normalized average expression of AMPA receptor GluA1 and GluA2 transcripts in B6.SJL mouse hippocampal neuron cultures exposed to full-length Aβ1–42 (Aβ42), the N-Aβcore (Aβ10–15) or the combination (combo), as measured via qPCR. Data are means ± SD. N = 3 cultures for each condition. ***p = 0.000001 (Bonferroni post hoc tests for one-way ANOVA comparison between Aβ42 and Aβ10–15). There was no statistical difference between expression for any condition for GluA2. (B) Expression of pAMPAR1 (pGluA1) or total AMPAR1 (GluA1) in the hippocampi of 5xFAD mice injected with sterile saline or N-Aβcore (Core), as measured using western immunoblot. N = 4 mice for each condition. Total GluA1: *p < 0.0001 (Chi-square).
Proteins solubilized from hippocampi isolated from 5xFAD mice bilaterally injected with the N-Aβcore or saline vehicle were assayed for changes in in vivo expression of hippocampal AMPAR1 (GluA1) via immunoblot analysis. Total GluA1 levels in the hippocampi were increased with exposure to the N-Aβcore as compared to saline-injected controls (Figure 2B; 248% ± 67% SD; p < 0.0001 by Chi-square (42.3, 3df). The increase in relative pAMPAR1 (pS831) in the hippocampi exposed to N-Aβcore was accounted by the increase in total GluA1 (Figure 2B; 127% ± 35% SD).
Structure-Function and Concentration-Dependence of the N-Aβcore in Reversing LTP Impairment in Hippocampal Slices From 5xFAD APP/PS1 Mice
We also tested for basic concentration-dependence of the N-Aβcore in reversing LTP impairment in the 5xFAD mouse hippocampal slices. Low concentration (0.5 pM) of the N-Aβcore showed no difference on LTP compared to control 5xFAD slices (Figures 1C,E; 107% ± 7% SD compared to 5xFAD).
Through Aβ-interacting receptor-linked Ca2+ and neurotoxicity assays, we had previously shown that mutating the tyrosine residue in the N-Aβcore to a serine [Y10S] or mutating the two histidine residues to two alanines [H13A, H14A] reduces activity, indicating these amino acid residues in the N-Aβcore sequence are essential for activity (Lawrence et al., 2014; Forest et al., 2018). To confirm the specificity of the N-Aβcore in reversing LTP impairment in 5xFAD hippocampal slices, we tested the inactive triple mutant [SEVAAQ] and the reverse-sequence N-Aβcore [QHHVEY]. Treatment with the reverse-sequence N-Aβcore partially restored LTP in the 5xFAD hippocampal slices (Figures 1D,E). Note that the reverse-sequence peptide contains the critical di-histidine sequence. By contrast, the inactive triple mutant had no significant effect on LTP in the 5xFAD slices (Figures 1D,E; 110% ± 13% SD compared to 5xFAD). It is important to note that there was no change in basal synaptic transmission or a trend toward increasing LTP in the wild-type slices treated with the reverse N-Aβcore (not shown), as seen for the N-Aβcore. Taken together, these results confirm the contribution of the two essential histidine residues to the neuromodulatory activity of the N-Aβcore.
N-Aβcore Rescue of Aβ-Induced LTP Deficits Involves PI3 Kinase and mTOR
Long-term potentiation has shown to involve multiple protein kinase and phosphatase pathways (Thomas and Huganir, 2004; Kennedy, 2013). As prior evidence implicates the PI3 kinase and mTOR pathways in the regulation of Aβ neurotoxicity (Lafay-Chebassier et al., 2005; Lee et al., 2008) and in the regulation of LTP (Hoeffer and Klann, 2010), we evaluated the roles of PI3 kinase and mTOR in the action of the N-Aβcore in reversing impaired LTP via treatment of the hippocampal slice preparations with selective inhibitors. As shown in Figure 3, application of PI3 kinase inhibitor LY294002 had no impact on baseline responses or LTP in hippocampal slices from control (background B6.SJL) mice or 5xFAD mice. In contrast, application of LY294002 prior to treatment with the N-Aβcore prevented the rescue by the N-Aβcore of LTP in the slices from 5xFAD mice (Figure 3; 94.3% ± 15% SD compared to 5xFAD).
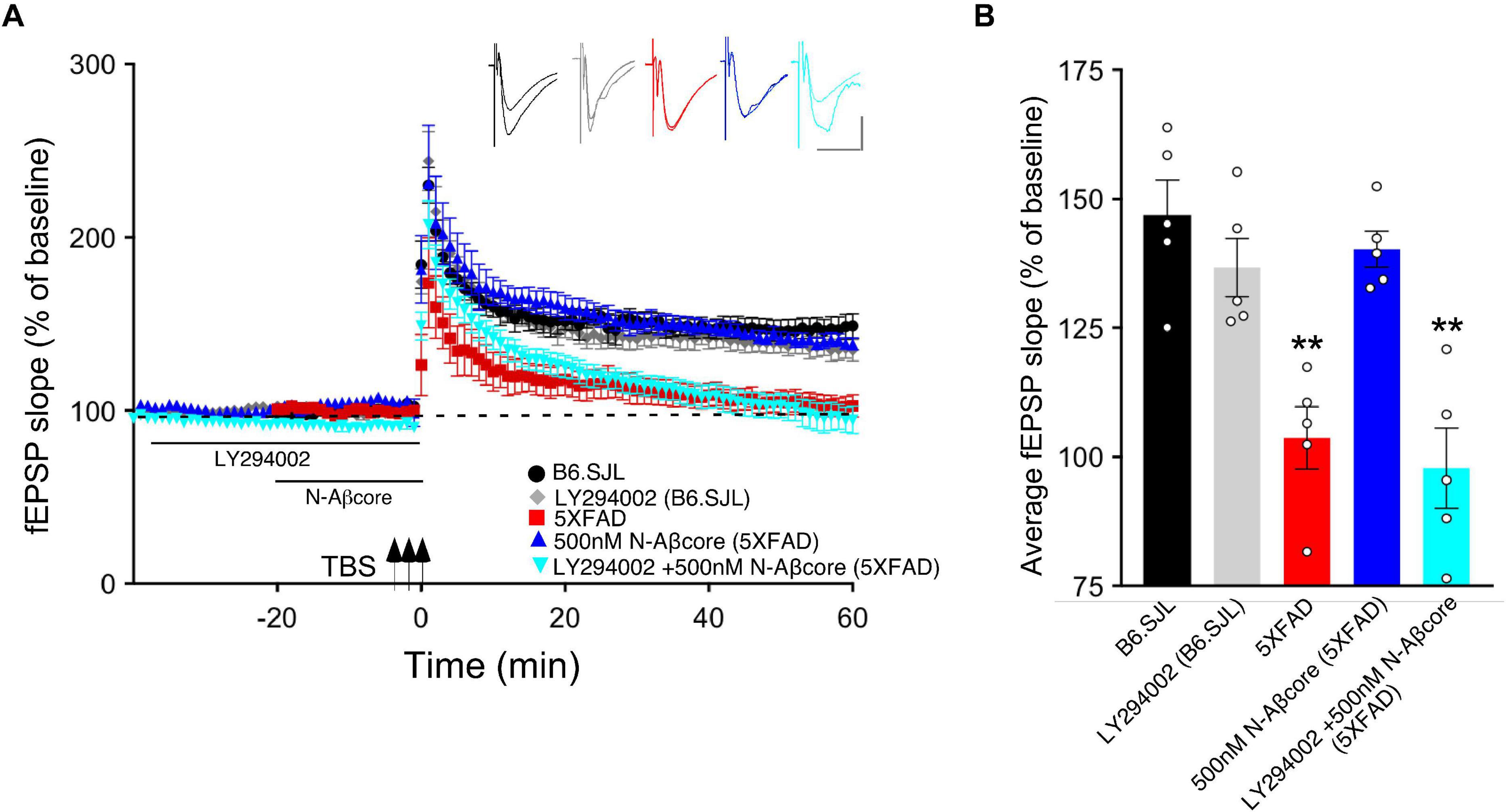
Figure 3. N-Aβcore protection of Aβ-induced synaptic dysfunction involves the PI3 kinase pathway. (A) TBS-induced LTP for B6.SJL slices perfused with control aCSF (black) or 100 μM LY294002 (gray) and for 5xFAD slices with aCSF (red), 500 nM N-Aβcore (blue) or 500 nM N-Aβcore plus 100μM LY294002 (cyan). Color-coded inserts showing examples of fEPSPs (baseline vs. LTP). (B) Quantification of average fEPSP slope values for 50–60 min post-TBS (one-way ANOVA: F(4,20) = 13.63, p < 0.0001). Bonferroni post hoc tests showed that rescue of the LTP deficit in the 500 nM N-Aβcore-treated 5xFAD slices (**p = 0.004, 500 nM N-Aβcore 5xFAD vs. 5xFAD; p < 0.05, vs. aCSF B6.SJL) was blocked by 100 μM LY294002 pretreatment (**p = 0.0002, 100μM LY294002 + 500 nM N-Aβcore vs. aCSF B6.SJL). Arrows indicate timing of TBS in (A). All data are expressed as means ± SEM. N = 4 for each condition (1 slice/mouse). Inset calibration: horizontal, 10 ms; vertical, 0.4 mV. **p < 0.005.
Prior inhibition of the PI3 kinase pathway effector mTOR by rapamycin also prevented the rescue by the N-Aβcore of LTP in the slices from 5xFAD mice (Figure 4B; 101% ± 20% SD compared to 5xFAD slices). The acute treatment with rapamycin had no significant effect on the control B6.SJL slices (Figure 4A). Rapamycin also had no significant effect on the reduced level of LTP in the 5xFAD slices at any point, and rapamycin prevented the rescue by N-Aβcore at all time points post-TBS. The inhibitor also did not affect baseline responses.
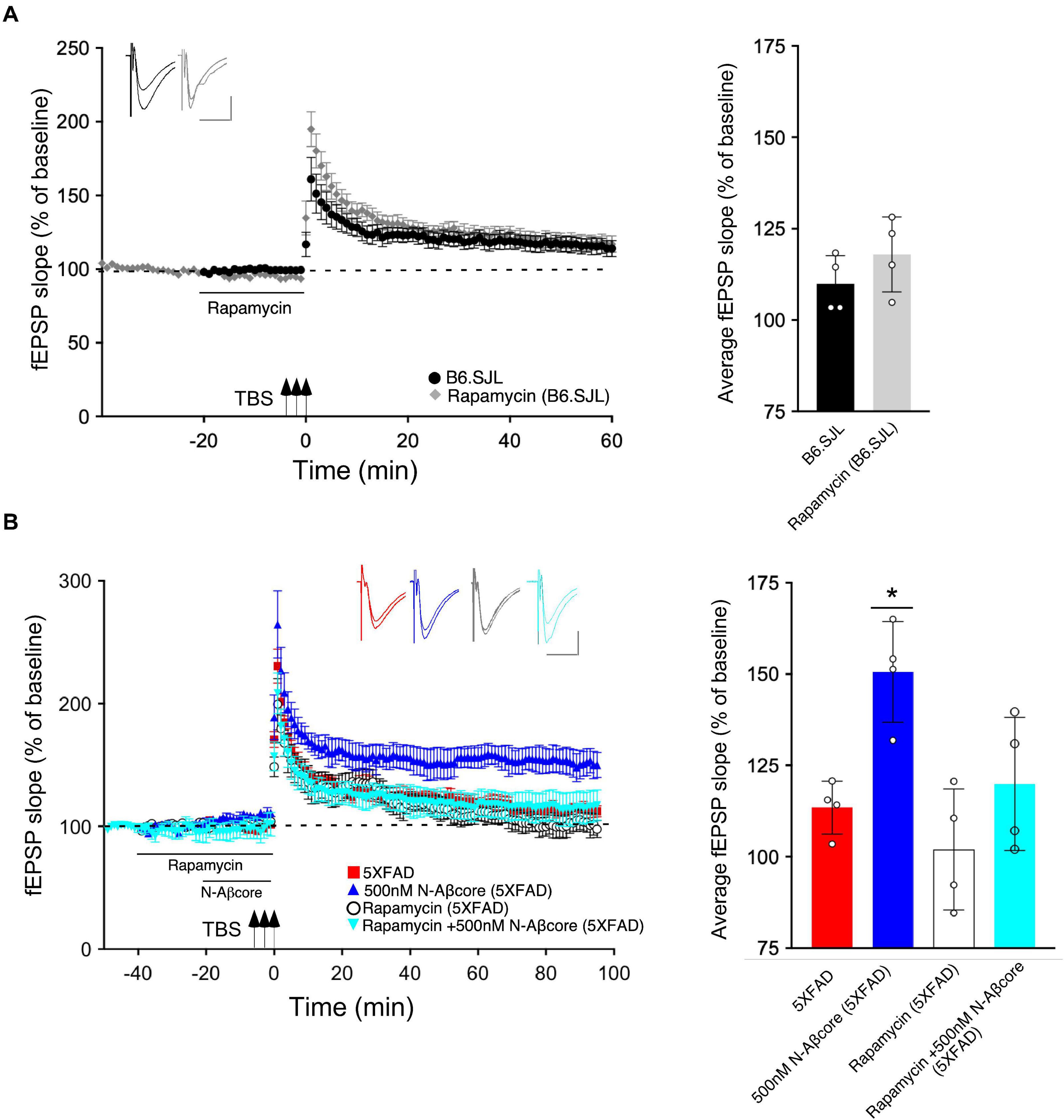
Figure 4. N-Aβcore protection of Aβ-induced synaptic dysfunction involves mTOR downstream in the PI3 kinase pathway. (A) Left, Lack of effect of acute pretreatment of 500 nM rapamycin (gray) on TBS-induced LTP for B6.SJL slices as compared to LTP induced in aCSF-treated B6.SJL control slices (black) from 4–5 month-old mice. Right, Comparison of average fEPSP slope values for 50–60 min post-TBS for B6.SJL slices incubated in 500 nM rapamycin to aCSF-treated B6.SJL control slices (t(6) = 0.26, p = 0.8023; two-tailed unpaired Student’s t-test). (B) Left, TBS-induced LTP for 5xFAD slices with aCSF (red), 500 nM rapamycin (white circle), 500 nM N-Aβcore (cyan) or 500 nM N-Aβcore plus 500 nM rapamycin (blue). Right, Rescue of LTP deficit following 20-min 500 nM N-Aβcore treatment in 5xFAD slices (*p = 0.0217, Bonferroni post hoc test after one-way ANOVA, F(3,12)) was abolished with 500 nM rapamycin pretreatment (40 min) (ns, Bonferroni: rapamycin + N-Aβcore in 5xFAD vs. 5xFAD alone). There was no effect of acute pretreatment of rapamycin on LTP in 5xFAD slices (ns, Bonferroni: rapamycin in 5xFAD vs. 5xFAD alone). Arrows indicate timing of TBS in (A) and (B). All recording data (A,B) are means ± SEM. Averaged data for 50–60 min post-tetanus are means ± SD. N = 4 for each condition (1 slice/mouse). Color-coded insets show examples of fEPSPs (baseline vs. LTP). Calibration bars for insets: horizontal, 10 ms; vertical, 0.4 mV.
To further probe the mechanism by which the N-Aβcore regulates the PI3 kinase pathway, the impact of the core peptide on Aβ-linked regulation of the PI3 kinase and its downstream effectors Akt and mTOR was investigated using mouse hippocampal neuron cultures. Treatment of neuron cultures with full-length Aβ (1–42) was shown to downregulate expression of various PI3 kinase, Akt and mTOR transcripts (Figure 5). While modest changes were noted on treatment with the N-Aβcore (not significant from control for mTOR), co-treatment with the N-Aβcore alleviated the Aβ-induced downregulation of Akt1 and mTOR transcripts (Figure 5).
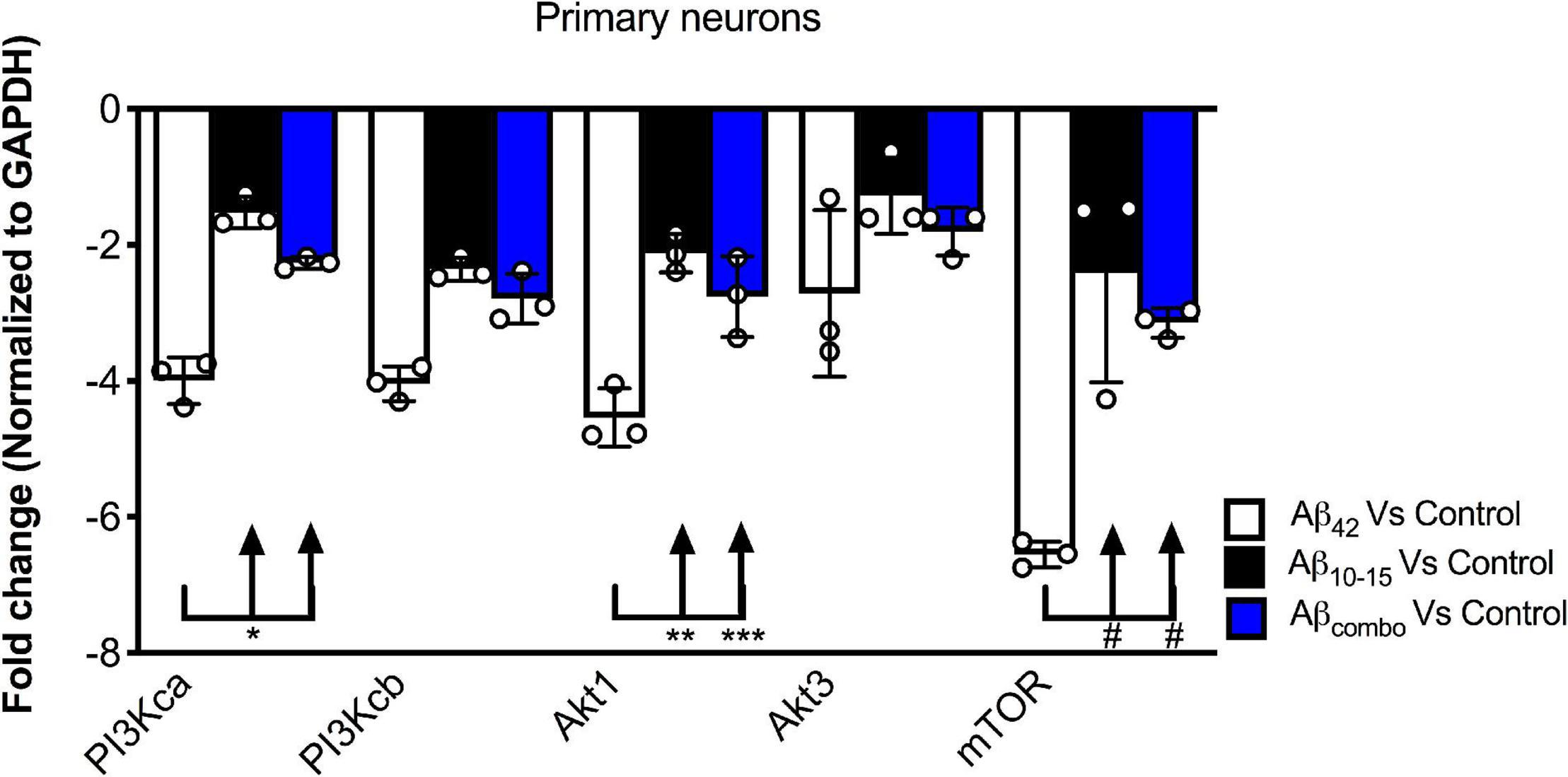
Figure 5. Impact of N-Aβcore on regulation of various PI3 kinase, Akt and mTOR transcripts by Aβ. Primary mouse hippocampal neuron cultures were treated with 1 μM full-length Aβ42, 1 μM Aβ10–15 (N-Aβcore) or the combination (combo). Extracted RNA was subjected to qPCR, probing for Class 1 PI3 kinase catalytic subunits (CA and CB), Akt1 and Akt2 and mTOR. Data are expressed as averaged fold-change over untreated controls, after normalizing for GAPDH expression. Bars are means ± SD. *p = 0.00063; **p = 0.000002; ***p = 0.0001; #p = 0.000001 (Bonferroni post hoc tests for one-way ANOVA comparisons, as shown). For each condition, N = 3 cultures.
Elevated Levels of Aβ Enhances LTD and the N-Aβcore Reverses Aβ-Linked LTD Enhancement in Hippocampal Slices From 5xFAD APP/PS1 Mice
Long-term depression is an essential component of synaptic plasticity underlying memory processing in the hippocampus, as synapses cycle between LTP and LTD, a process known as synaptic scaling (Nägerl et al., 2004). To date, few studies have examined the effects of pathological levels of soluble Aβ on LTD induction, and moreover, the results have been mixed. For example, while focusing on NMDA receptor-dependent LTD, administration of synthetic Aβ led to an enhancement of LTD in some cases (e.g., Kim et al., 2001; Shankar et al., 2008; Li et al., 2009), whereas other studies reported no effect (e.g., Wang et al., 2002). Here, we aimed to examine the effects of endogenous soluble Aβ on LTD in the 5xFAD hippocampal slices. Using LFS to induce LTP in the same Schaffer collateral – CA1 pathway in hippocampal slice as that used for LTP, the LTD in slices from the 5xFAD mice was more pronounced than that observed for LTD induced in slices from B6.SJL control mice (Figures 6A,B; −32.5% ± −5.1% SD for 5xFAD compared to −18.1% ± −4.9% SD for the control B6.SJL slices). Interestingly, treatment of 5xFAD mouse slices with the N-Aβcore prior to and during LFS resulted in a restoration of LTD to the level observed for the B6.SJL slices (Figures 6A,B; −21.4% ± 4.5%). The N-Aβcore had no effect on metabotropic glutamate receptor-induced LTD (Figures 6C,D). Taken together, these data suggest that Aβ plays a role in facilitating LTD and the N-Aβcore may protect against Aβ-induced LTD enhancement. The role of the NMDA receptor in Aβ facilitation of LTD warrants further investigation.
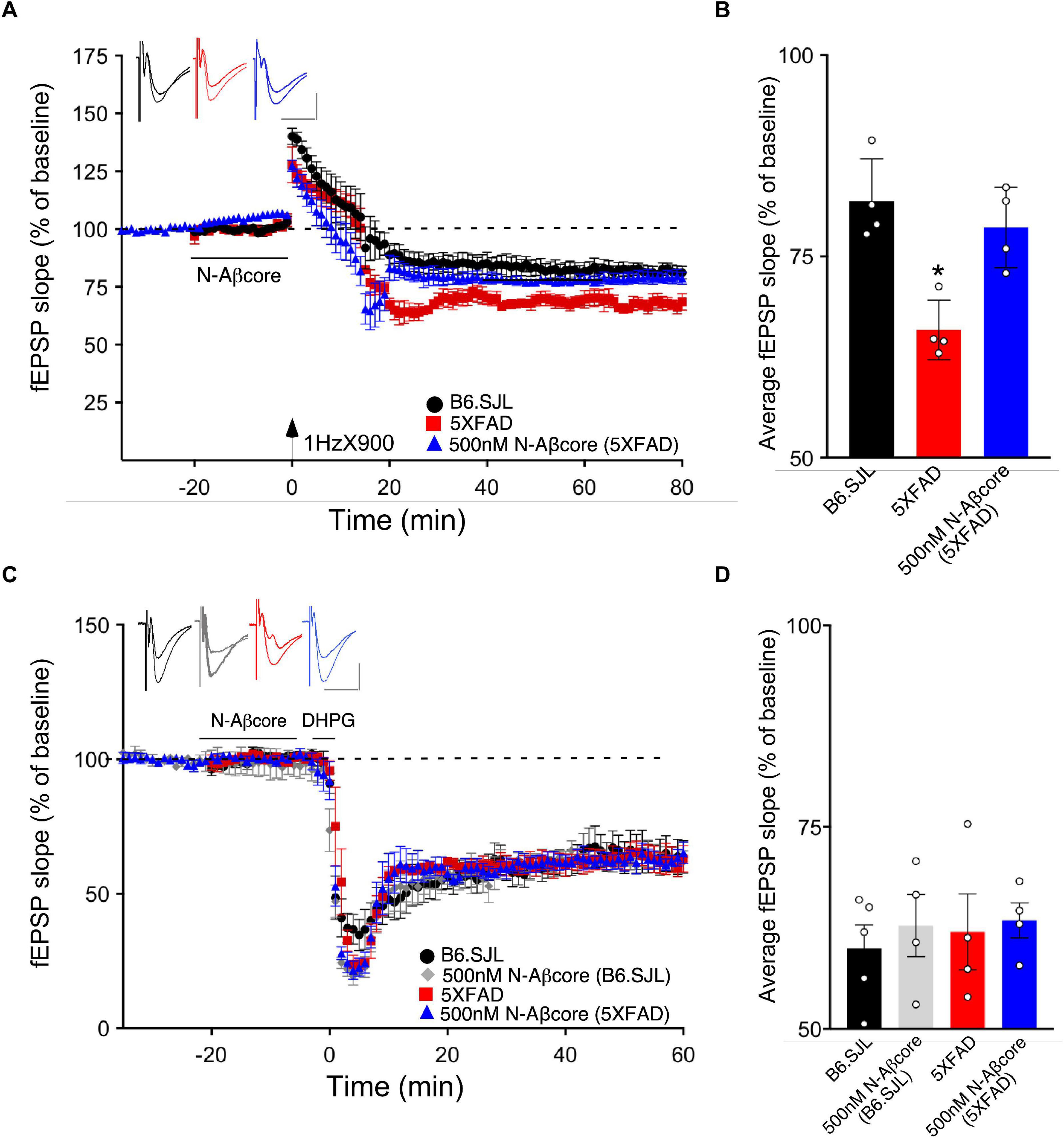
Figure 6. The N-Aβcore reverses endogenous Aβ enhancement of LTD, but has no impact on NMDA-independent, metabotropic glutamate receptor-induced LTD deficits. (A) LFS-induced LTD in hippocampal slices from B6.SJL perfused with control aCSF (black) and 5xFAD slices perfused with aCSF (red) or 500 nM N-Aβcore (blue). Color-coded insets show examples of fEPSPs (baseline vs. LTD). (B) Quantification of average fEPSP slope values for 50–60 min post-LFS (one-way ANOVA F(2,9) = 13.03, p = 0.0022). Bonferroni post hoc tests: 5xFAD slices displayed significantly more pronounced LTD as compared to control aCSF B6.SJL slices (*p = 0.012). 500 nM N-Aβcore in 5xFAD slices returned LTD to the level observed in control aCSF-perfused B6.SJL slices (p > 0.99). Data are mean ± SEM. N = 4 for each condition (1 slice/mouse). Inset calibration: horizontal, 10 ms; vertical, 0.4 mV. *p < 0.05. (C) NMDA-independent, 3,5-dihydroxyphenylglycine (DHPG, metabotropic glutamate receptor group I agonist; 100 μM)-induced LTD in hippocampal slices from B6.SJL mice perfused with control aCSF (black) or 500 nM N-Aβcore (gray) and 5xFAD slices perfused with aCSF (red) or 500 nM N-Aβcore (blue). Color-coded insets show examples of fEPSPs (baseline vs. LTD). Inset calibration: horizontal, 10 ms; vertical, 0.4 mV. (D) Quantification of average fEPSP slope values for 50–60 min post-LFS (p < 0.05, two-way ANOVA). Data are means ± SEM. N = 4 for each condition (1 slice/mouse).
Discussion
Previous studies have established a strong link between the progression of AD and the extent of synaptic dysfunction occurring in the early stages of the disease, prior to the formation of Aβ plaques and tau neurofibrillary tangles (Terry et al., 1991; Scheff et al., 2006; Shankar and Walsh, 2009; Koffie et al., 2011). In AD-endophenotype APP transgenic mouse models, though limited in regard to physiological context for APP expression, it has been widely demonstrated that elevated levels of soluble oligomeric Aβ drive LTP inhibition (Cullen et al., 1997; Chapman et al., 1999; Walsh et al., 2002; Kimura and Ohno, 2009), coupled to downregulation of synaptic AMPARs. By contrast, the link between pathological levels of Aβ and LTD are less well understood, and as previously noted, investigations of the impact of Aβ on LTD have had conflicting results.
Low “physiological” levels (pM) of soluble Aβ have been shown to enhance synaptic plasticity and facilitate hippocampal-based learning and memory (Puzzo et al., 2008; Puzzo et al., 2012; Lawrence et al., 2014), suggesting a neuromodulatory role of soluble Aβ at physiological levels. Augmentation of LTP by pM Aβ correlated with enhanced expression of AMPARs. Similar results obtained using an N-terminal fragment of Aβ (1–15) implicated that sequence within Aβ as accountable for the positive neuromodulatory activity of full-length Aβ (Lawrence et al., 2014). We wondered whether the N-Aβcore (10–15; YEVHHQ), encompassing the essential sequence within the N-terminal fragment accounting for its positive neuromodulatory activity and its cellular neuroprotective activity against Aβ neurotoxicity, could itself enhance synaptic plasticity and protect against Aβ-induced synaptic dysfunction.
In accordance with previous findings (Kimura and Ohno, 2009), we found that LTP was nearly absent in the hippocampal slice model from APP/PS1 5xFAD transgenic mice, previously shown to be accounted by elevated Aβ in the brains of the transgenic model mice (Oakley et al., 2006; Crouzin et al., 2013), though the possibility of altered levels of the different sAPP variants in 5xFAD mice is to be noted and, while primarily neurotrophic (see Dar and Glazner, 2020), they may also contribute to the mutant phenotype and regulation of synaptic plasticity by the N-Aβcore. Treatment here with the N-Aβcore reversed this deficit back to the LTP observed in slices from age-matched background B6.SJL mice. Treatment of 5xFAD slices with the N-Aβcore trended toward LTP enhancement, suggesting that the reversal of the LTP deficits in the 5xFAD slices by the N-Aβcore was not solely due to competitive binding for target receptors and may possibly involve activation of a neuroprotective pathway that enhances synaptic plasticity (see Forest and Nichols, 2019). Here, we identified the PI3 kinase/Akt/mTOR in the reversal of LTP deficits in 5xFAD slices by the N-Aβcore as a primary pathway, which has been shown to be a key link to long-term memory (Bekinschtein et al., 2007). Under the conditions used for the mutant APP/PS1 5xFAD model preparation, we did not observe any evident impact of the mTOR inhibitor rapamycin, consistent with existing mTOR dysregulation of LTP in AD model mouse preparations (Ma et al., 2010), shown to be rescued by upregulation of mTOR. Our findings using selective inhibitors suggest that treatment of the 5xFAD slice preparations with the N-Aβcore upregulated PI3 kinase/Akt/mTOR, accounting, at least in part, for the rescue by the N-Aβcore of hippocampal LTP deficits in this mutant APP/PS1 5xFAD model.
Other downstream pathways engaged by the N-Aβcore are not yet definitely identified, but we suspect that key players involved in synaptic modulation may also affected, such as regulation of CREB, PKA, and/or CAMKII or downregulation of calcineurin and/or PP1, subsequently altering AMPA receptor trafficking to the synapses (Derkach et al., 2007; Makino and Malinow, 2009; Huganir and Nicoll, 2013; Herring and Nicoll, 2016). Additionally, the enhancement of the basal synaptic transmission with the treatment of the N-Aβcore suggests a receptor-linked influx of Ca2+, which further supports the idea that the N-Aβcore activates an alternative neuroprotective pathway that enhances synaptic plasticity, consistent with results for neuroprotection by the N-Aβcore in Aβ-triggered neurotoxicity (Forest et al., 2018). Previously, it has been shown that BDNF enhances basal synaptic transmission (Patterson et al., 1996), and therefore, it may be that the N-Aβcore-induced Ca2+ influx could regulate BDNF release at the synapses, thus enhancing baseline synaptic transmission and ultimately LTP (see Lu, 2003). It would be interesting to examine the effect of the basal synaptic transmission by the N-Aβcore long-term, and whether the enhancement of LTP observed involved BDNF.
In the context of synaptic plasticity, LTD is necessary for neural homeostasis. NMDA receptor-dependent LTD involves internalization of AMPA receptors via a caspase-dependent pathway (D’Amelio et al., 2011; Li and Sheng, 2012). To date, however, there is limited understanding in regard to the effects of pathological Aβ on LTD, where some groups show that synthetic Aβ enhances LTD (Kim et al., 2001; Shankar et al., 2008; Hu et al., 2014) and others show no effect (Wang et al., 2002; Raymond et al., 2003), though the differential action of Aβ may have resulted from recording in different subregions of the hippocampus (e.g., CA1 vs. dentate gyrus). Here, we found that high concentrations of endogenous soluble Aβ shown to be present in the brains of 5xFAD mice resulted in enhanced LTD in hippocampal slices, and treatment with effective concentrations of N-Aβcore prior to and during the LFS induction of LTD reverses this enhancement. Interestingly, Hu et al. (2014) found that applying synthetic soluble Aβ prior to LFS did not affect the early phase of LFS-induced LTD (<2 h post LFS), but facilitated the late phase (3–5 h post LFS), thus, possibly accounting for different findings. It is important to note that late-phase LTP and LTD require new protein synthesis, and mTOR is linked to the regulation of protein synthesis (Wang and Proud, 2006). Indeed, as previously noted, the reversal by the N-Aβcore of LTP deficit in the 5xFAD slices was dependent upon mTOR. As LTD and LTP work in concert to allow for reversible synaptic plasticity and synaptic scaling, the LFS-induced enhancement of LTD in the 5xFAD slices could affect subsequent LTP, and this may be another reason why an LTP deficit was observed in the 5xFAD slices compared to wild-type preparations.
Although NMDA and AMPA receptors are involved in different aspects of LTP and LTD, and their expression is affected by elevated Aβ, metabotropic glutamate receptors (mGluRs) have also been implicated in Aβ-induced synaptic dysfunction (Wang et al., 2004; Chen et al., 2013; Hu et al., 2014; Haas et al., 2016). Interestingly, NMDA-independent, mGluR-induced LTD was found to be unaffected by the N-Aβcore. While mGluRs have been linked via cellular prion to Aβ-induced cellular toxicity (Haas et al., 2016), our findings support a divergence in the Aβ-linked signaling pathways affected by the N-Aβcore in synaptic plasticity. Further work is needed to elucidate the detailed molecular mechanisms involved in N-Aβcore protection or reversal of Aβ-linked synaptic dysfunction, including caspase-dependent intracellular pathways leading to the regulation of AMPA receptors, and eventual synaptic loss in AD.
Conclusion
The essential core hexapeptide sequence, YEVHHQ, or N-Aβcore, within the neuroprotective N-terminal fragment of Aβ was able to effectively, selectively and potently reverse deficits in synaptic plasticity in hippocampal slices from adult APP/PS1 5xFAD transgenic mice. Attenuated LTP and enhanced LTD observed in the slices from 5xFAD mice were both rescued by the N-Aβcore, returning LTP and LTD back to the levels found for hippocampal slices from background control (B6.SJL) mice. The involvement of the PI3 kinase pathway, specifically, mTOR in the protective action of the N-Aβcore against FAD-linked deficits in synaptic plasticity indicates possible connection between N-Aβcore-linked signaling and the translation machinery at hippocampal synaptic sites (dendritic spines). It would thus be of interest to examine the specific signaling pathways engaged by N-Aβcore at hippocampal sites, especially key downstream elements in translational control such as S6 kinase, a prominent substrate for mTORC1 in neuronal systems.
Data Availability Statement
The datasets generated for the current study are available from the corresponding author on reasonable request.
Ethics Statement
All experiments using animals were performed under approved University of Hawai‘i Institutional Animal Care and Use Committee (IACUC) protocols.
Author Contributions
KF and RN contributed to the conceptualization and writing – original draft. KF, RT, KA, and CT contributed to the methodology. KF, RT, KA, CT, and RN contributed to the data analysis. KF, RT, CT, and RN contributed to writing – review and editing. All authors contributed to the article and approved the submitted version.
Funding
RN was supported by the University of Hawai‘i Foundation, #125-8320-4.
Conflict of Interest
The authors declare that the research was conducted in the absence of any commercial or financial relationships that could be construed as a potential conflict of interest.
Acknowledgments
We thank Dr. Naghum Alfulaij for advice. We thank Ms. Ann Hashimoto for mouse breeding and husbandry. We thank Dr. Tessi Sherrin for help with the hippocampal microinjections. This manuscript has been released as a pre-print at bioRxiv 2020.03.17.995191 (doi: https://doi.org/10.1101/2020.03.17.995191).
References
Bekinschtein, P., Katche, C., Slipczuk, L. N., Igaz, L. M., Cammarota, M., Izquierdo, I., et al. (2007). mTOR signaling in the hippocampus is necessary for memory formation. Neurobiol. Learn. Mem. 87, 303–307. doi: 10.1016/j.nlm.2006.08.007
Chapman, P. F., White, G. L., Jones, M. W., Cooper-Blacketer, D., Marshall, V. J., Irizarry, M., et al. (1999). Impaired synaptic plasticity and learning in aged amyloid precursor protein transgenic mice. Nat. Neurosci. 2, 271–276. doi: 10.1038/6374
Chen, X., Lin, R., Chang, L., Xu, S., Wei, X., Zhang, J., et al. (2013). Enhancement of long-term depression by soluble amyloid β protein in rat hippocampus is mediated by metabotropic glutamate receptor and involves activation of p38MAPK, STEP and caspase-3. Neuroscience 253, 435–443. doi: 10.1016/j.neuroscience.2013.08.054
Cheng, Q., and Yakel, J. L. (2015). Activation of α7 nicotinic acetylcholine receptors increases intracellular cAMP levels via activation of AC1 in hippocampal neurons. Neuropharmacol 95, 405–414. doi: 10.1016/j.neuropharm.2015.04.016
Crouzin, N., Baranger, K., Cavalier, M., Marchalant, Y., Cohen-Solal, C., Roman, F. S., et al. (2013). Area-specific alterations of synaptic plasticity in the 5XFAD mouse model of Alzheimer’s disease: dissociation between somatosensory cortex and hippocampus. PLoS One 8:e74667. doi: 10.1371/journal.pone.0074667
Cullen, W. K., Suh, Y. H., Anwyl, R., and Rowan, M. J. (1997). Block of LTP in rat hippocampus in vivo by β-amyloid precursor protein fragments. Neuroreport 8, 3213–3217. doi: 10.1097/00001756-199710200-00006
D’Amelio, M., Cavallucci, V., Middei, S., Marchetti, C., Pacioni, S., Ferri, A., et al. (2011). Caspase-3 triggers early synaptic dysfunction in a mouse model of Alzheimer’s disease. Nat. Neurosci. 14, 69–76. doi: 10.1038/nn.2709
Dar, N. J., and Glazner, G. W. (2020). Deciphering the neuroprotective and neurogenic potential of soluble amyloid precursor protein alpha (sAPPα). Cell. Mol. Life Sci. 77, 2315–2330. doi: 10.1007/s00018-019-03404-x
Derkach, V. A., Oh, M. C., Guire, E. S., and Soderling, T. R. (2007). Regulatory mechanisms of AMPA receptors in synaptic plasticity. Nat. Rev. Neurosci. 8, 101–113. doi: 10.1038/nrn2055
Forest, K. H., Alfulaij, N., Arora, K., Taketa, R., Sherrin, T., Todorovic, C., et al. (2018). Protection against β-amyloid neurotoxicity by a non-toxic endogenous N-terminal β-amyloid fragment and its active hexapeptide core sequence. J. Neurochem. 144, 201–217. doi: 10.1111/jnc.14257
Forest, K. H., and Nichols, R. A. (2019). Assessing neuroprotective agents for Aβ-induced neurotoxicity. Trends Mol. Med. 25, 685–695. doi: 10.1016/j.molmed.2019.05.013
Forest, K. H., Taketa, R., Arora, K., Todorovic, C., and Nichols, R. A. (2020). The neuroprotective beta amyloid hexapeptide core reverses deficits in synaptic plasticity in the 5×FAD APP/PS1 mouse model (preprint). bioRxiv 2020:995191. doi: 10.1101/2020.03.17.995191
Glabe, C. C. (2005). ““Amyloid accumulation and pathogenesis of Alzheimer’s disease: significance of monomeric, oligomeric and fibrillar Aβ”,” in Alzheimer’s disease: Cellular and molecular aspects of amyloid β, eds J. R. Harris and F. Fahrenholz (Boston, MA: Springer), 167–177.
Gulisano, W., Melone, M., Ripoli, C., Tropea, M. R., Li Puma, D. D., Giunta, S., et al. (2019). Neuromodulatory action of picomolar extracellular Aβ42 oligomers on presynaptic and postsynaptic mechanisms underlying synaptic function and memory. J. Neurosci. 39, 5986–6000. doi: 10.1523/JNEUROSCI.0163-19.2019
Haas, L. T., Salazar, S. V., Kostylev, M. A., Um, J. W., Kaufman, A. C., and Strittmatter, S. M. (2016). Metabotropic glutamate receptor 5 couples cellular prion protein to intracellular signaling in Alzheimer’s disease. Brain 139, 526–546. doi: 10.1093/brain/awv356
Herring, B. E., and Nicoll, R. A. (2016). Long-term potentiation: from CaMKII to AMPA receptor trafficking. Annu. Rev. Physiol. 78, 351–365. doi: 10.1146/annurev-physiol-021014-071753
Hoeffer, C. A., and Klann, E. (2010). mTOR signaling: at the crossroads of plasticity, memory and disease. Trends Neurosci. 33, 67–75. doi: 10.1016/j.tins.2009.11.003
Hou, L., and Klann, E. (2004). Activation of the phosphoinositide 3-kinase-Akt-mammalian target of rapamycin signaling pathway is required for metabotropic glutamate receptor-dependent long-term depression. J. Neurosci. 24, 6352–6361. doi: 10.1523/JNEUROSCI.0995-04.2004
Hsieh, H., Boehm, J., Sato, C., Iwatsubo, T., Tomita, T., Sisodia, S., et al. (2006). AMPAR removal underlies Aβ-induced synaptic depression and dendritic spine loss. Neuron 52, 831–843. doi: 10.1016/j.neuron.2006.10.035
Hu, N.-W., Nicoll, A. J., Zhang, D., Mably, A. J., O’Malley, T., Purro, S. A., et al. (2014). mGlu5 receptors and cellular prion protein mediate amyloid-β-facilitated synaptic long-term depression in vivo. Nat. Commun. 5:3374. doi: 10.1038/ncomms4374
Huganir, R. L., and Nicoll, R. A. (2013). AMPARs and synaptic plasticity: the last 25 years. Neuron 80, 704–717. doi: 10.1016/j.neuron.2013.10.025
Kennedy, M. B. (2013). Synaptic signaling in learning and memory. Cold Spring Harb. Perspect. Biol. 8:a016824. doi: 10.1101/cshperspect.a016824
Kim, J.-H., Anwyl, R., Suh, Y.-H., Djamgoz, M. B. A., and Rowan, M. J. (2001). Use-dependent effects of amyloidogenic fragments of β-amyloid precursor protein on synaptic plasticity in rat hippocampus in vivo. J. Neurosci. 21, 1327–1333. doi: 10.1523/JNEUROSCI.21-04-01327.2001
Kimura, R., and Ohno, M. (2009). Impairments in remote memory stabilization precede hippocampal synaptic and cognitive failures in 5XFAD Alzheimer mouse model. Neurobiol. Dis. 33, 229–235. doi: 10.1016/j.nbd.2008.10.006
Koffie, R. M., Hyman, B. T., and Spires-Jones, T. L. (2011). Alzheimer’s disease: synapses gone cold. Mol. Neurodegener. 6:63. doi: 10.1186/1750-1326-6-63
Lafay-Chebassier, C., Paccalin, M., Page, G., Barc-Pain, S., Perault-Pochat, M. C., Gil, R., et al. (2005). mTOR/p70S6k signaling alteration by Aβ exposure as well as in APP-PS1 transgenic models and in patients with Alzheimer’s disease. J. Neurochem. 94, 215–225. doi: 10.1111/j.1471-4159.2005.03187.x
Lawrence, J. L. M., Tong, M., Alfulaij, N., Sherrin, T., Contarino, M., White, M. M., et al. (2014). Regulation of presynaptic Ca2+, synaptic plasticity and contextual fear conditioning by a N-terminal β-amyloid fragment. J. Neurosci. 34, 14210–14218. doi: 10.1523/JNEUROSCI.0326-14.2014
Lee, K.-Y., Koh, S.-H., Noh, M. Y., Kim, S. H., and Lee, Y. J. (2008). Phosphatidylinositol-3-kinase activation blocks amyloid beta-induced neurotoxicity. Toxicology 243, 43–50. doi: 10.1016/j.tox.2007.09.020
Li, S., Hong, S., Shepardson, N. E., Walsh, D. M., Shankar, G. M., and Selkoe, D. (2009). Soluble oligomers of amyloid β-protein facilitate hippocampal long-term depression by disrupting neuronal glutamate uptake. Neuron 62, 788–801. doi: 10.1016/j.neuron.2009.05.012
Li, Z., and Sheng, M. (2012). Caspases in synaptic plasticity. Mol. Brain 5:15. doi: 10.1186/1756-6606-5-15
Lu, B. (2003). BDNF and activity-dependent synaptic modulation. Learn. Mem. 10, 86–98. doi: 10.1101/lm.54603
Ma, T., Hoeffer, C. A., Capetillo-Zarate, E., Yu, F., Wong, H., Lin, M. T., et al. (2010). Dysregulation of the mTOR pathway mediates impairment of synaptic plasticity in a mouse model of Alzheimer’s disease. PLoS One 5:e12845. doi: 10.1371/journal.pone.0012845
Makino, H., and Malinow, R. (2009). AMPA receptor incorporation into synapses during LTP: the role of lateral movement and exocytosis. Neuron 64, 381–390. doi: 10.1016/j.neuron.2009.08.035
Morrisey, J. A., Mockett, B. G., Singh, A., Kweon, D., Ohline, S. M., Tate, W. P., et al. (2019). A C-terminal peptide from secreted amyloid precursor protein-α enhances long-term potentiation in rats and a transgenic mouse model of Alzheimer’s disease. Neuropharmacology 157:107670. doi: 10.1016/j.neuropharm.2019.107670
Nägerl, U. V., Eberhorn, N., Cambridge, S. B., and Bonhoeffer, T. (2004). Bidirectional activity-dependent morphological plasticity in hippocampal neurons. Neuron 44, 759–767. doi: 10.1016/j.neuron.2004.11.016
Oakley, H., Cole, S. L., Logan, S., Maus, E., Shao, P., Craft, J., et al. (2006). Intraneuronal β-amyloid aggregates, neurodegeneration, and neuron loss in transgenic mice with five familial Alzheimer’s disease mutations: potential factors in amyloid plaque formation. J. Neurosci. 26, 10129–10140. doi: 10.1523/JNEUROSCI.1202-06.2006
Oddo, S., Caccamo, A., Shepherd, J. D., Murphy, M. P., Golde, T. E., Kayed, R., et al. (2003). Triple-transgenic model of Alzheimer’s disease with plaques and tangles: intracellular Aβ and synaptic dysfunction. Neuron 39, 409–421. doi: 10.1016/S0896-6273(03)00434-3
Patterson, S. L., Abel, T., Deuel, T. A., Martin, K. C., Rose, J. C., and Kandel, E. R. (1996). Recombinant BDNF rescues deficits in basal synaptic transmission and hippocampal LTP in BDNF knockout mice. Neuron 16, 1137–1145. doi: 10.1016/s0896-6273(00)80140-3
Portelius, E., Price, E., Brinkmalm, G., Stieler, M., Olsson, M., Persson, R., et al. (2011). A novel pathway for amyloid precursor processing. Neurobiol. Aging 32, 1090–1098. doi: 10.1016/j.neurobiolaging.2009.06.002
Puzzo, D., Privitera, L., Leznik, E., Fà, M., Staniszewski, A., Palmeri, A., et al. (2008). Picomolar amyloid-β positively modulates synaptic plasticity and memory in hippocampus. J. Neurosci. 28, 14537–14545. doi: 10.1523/JNEUROSCI.2692-08.2008
Puzzo, D., Privitera, L., and Palmeri, A. (2012). Hormetic effect of amyloid-β peptide in synaptic plasticity and memory. Neurobiol. Aging 33, e15–e24. doi: 10.1016/j.neurobiolaging.2011.12.020
Raymond, C. R., Ireland, D. R., and Abraham, W. C. (2003). NMDA receptor regulation by amyloid-β does not account for its inhibition of LTP in rat hippocampus. Brain Res. 968, 263–272. doi: 10.1016/s0006-8993(03)02269-8
Reddy, P. H., Mani, G., Park, B. S., Jacques, J., Murdoch, G., Whetsell, W., et al. (2005). Differential loss of synaptic proteins in Alzheimer’s disease: implications for synaptic dysfunction. J. Alzheimers Dis. 7, 103–117. doi: 10.3233/JAD-2005-7203
Scheff, S. W., Price, D. A., Schmitt, F. A., and Mufson, E. J. (2006). Hippocampal synaptic loss in early Alzheimer’s disease and mild cognitive impairment. Neurobiol. Aging 27, 1372–1384. doi: 10.1016/j.neurobiolaging.2005.09.012
Shankar, G. M., Li, S., Mehta, T. H., Garcia-Munoz, A., Shepardson, N. E., Smith, I., et al. (2008). Amyloid β-protein dimers isolated directly from Alzheimer’s brains impair synaptic plasticity and memory. Nat. Med. 14, 837–842. doi: 10.1038/nm1782
Shankar, G. M., and Walsh, D. M. (2009). Alzheimer’s disease: synaptic dysfunction and Aβ. Mol. Neurodegener. 4:48. doi: 10.1186/1750-1326-4-48
Snyder, E. M., Nong, Y., Almeida, C. G., Paul, S., Moran, T., Choi, E. Y., et al. (2005). Regulation of NMDA receptor trafficking by amyloid-β. Nat. Neurosci. 8, 1051–1058. doi: 10.1038/nn1503
Terry, R. D., Masliah, E., Salmon, D. P., Butters, N., DeTeresa, R., Hill, R., et al. (1991). Physical basis of cognitive alterations in Alzheimer’s disease: synapse loss is the major correlate of cognitive impairment. Ann. Neurol. 30, 572–580. doi: 10.1002/ana.410300410
Thomas, G. M., and Huganir, R. L. (2004). MAPK cascade signalling and synaptic plasticity. Nat. Rev. Neurosci. 5, 173–183. doi: 10.1038/nrn1346
Walsh, D. M., Klyubin, I., Fadeeva, J. V., Cullen, W. K., Anwyl, R., Wolfe, M. S., et al. (2002). Naturally secreted oligomers of amyloid β protein potently inhibit hippocampal long-term potentiation in vivo. Nature 416, 535–539. doi: 10.1038/416535a
Wang, H.-W., Pasternak, J. F., Kuo, H., Ristic, H., Lambert, M. P., Chromy, B., et al. (2002). Soluble oligomers of β amyloid (1-42) inhibit long-term potentiation but not long-term depression in rat dentate gyrus. Brain Res. 924, 133–140. doi: 10.1016/s0006-8993(01)03058-x
Wang, Q., Walsh, D. M., Rowan, M. J., Selkoe, D. J., and Anwyl, R. (2004). Block of long-term potentiation by naturally secreted and synthetic amyloid β-peptide in hippocampal slices is mediated via activation of the kinases c-Jun N-terminal kinase, cyclin-dependent kinase 5, and p38 mitogen-activated protein kinase as well as metabotropic glutamate receptor type 5. J. Neurosci. 24, 3370–3378. doi: 10.1523/JNEUROSCI.1633-03.2004
Keywords: beta amyloid (Aβ), neuroprotection, Alzheimer’s disease, hippocampal slice, long-term potentiation, long-term depression
Citation: Forest KH, Taketa R, Arora K, Todorovic C and Nichols RA (2021) The Neuroprotective Beta Amyloid Hexapeptide Core Reverses Deficits in Synaptic Plasticity in the 5xFAD APP/PS1 Mouse Model. Front. Mol. Neurosci. 14:576038. doi: 10.3389/fnmol.2021.576038
Received: 25 June 2020; Accepted: 11 March 2021;
Published: 12 April 2021.
Edited by:
Oliver Wirths, University Medical Center Göttingen, GermanyReviewed by:
Igor Klyubin, Trinity College Dublin, IrelandTakashi Saito, Nagoya City University, Japan
Copyright © 2021 Forest, Taketa, Arora, Todorovic and Nichols. This is an open-access article distributed under the terms of the Creative Commons Attribution License (CC BY). The use, distribution or reproduction in other forums is permitted, provided the original author(s) and the copyright owner(s) are credited and that the original publication in this journal is cited, in accordance with accepted academic practice. No use, distribution or reproduction is permitted which does not comply with these terms.
*Correspondence: Robert A. Nichols, robert.nichols@hawaii.edu; orcid.org/0000-0003-0366-0162
†Present address: Kelly H. Forest, Department of Biology, Dartmouth College, Hanover, NH, United States; Komal Arora, Department of Biology, College of Arts and Sciences, Georgia State University, Atlanta, GA, United States