- 1College of Animal Science and Technology, Hunan Agricultural University, Changsha, China
- 2Key Laboratory of Agro-Ecological Processes in Subtropical Region, Hunan Provincial Engineering Research Center of Healthy Livestock, Scientific Observing and Experimental Station of Animal Nutrition and Feed Science in South-Central, Ministry of Agriculture, Institute of Subtropical Agriculture, Chinese Academy of Sciences, Changsha, China
- 3Hunan Collaborative Innovation Center for Utilization of Botanical Functional Ingredients and Hunan Collaborative Innovation Center of Animal Production Safety, Changsha, China
- 4College of Animal Science and Technology, Northwest A&F University, Yangling, China
Alpha-ketoglutarate (AKG), a critical molecule in the tricarboxylic acid cycle, is beneficial to intestinal functions. However, its influence on intestinal microbiota and metabolism is not fully understood. We investigated the effects of a low-protein (LP) diet supplemented with AKG on cecal microbial communities and the parameters of microbial metabolism in growing pigs. Twenty-seven young pigs (Large White × Landrace) with an average initial body weight of 11.96 ± 0.18 kg were randomly allotted into three groups (n = 9): a normal protein (NP) diet containing 20% crude protein (CP); LP diet formulated with 17% CP (LP diet); or LP diet supplemented with 10 g kg-1 of AKG (ALP diet). After a 35-day trial period, the digesta of the cecum were collected to analyze the concentrations of ammonia and short-chain fatty acids (SCFAs). We also performed a microbial analysis. Although no significant differences were found in performance among the diet groups, pigs fed the ALP diet had greater average daily gain (ADG) when compared with those in the LP group. Experimental diet did not affect cecal bacterial richness or diversity, as determined by Chao1 and ACE species richness measures and Shannon and Simpson indices, respectively. The predominant phyla Firmicutes, Bacteroidetes, and Proteobacteria increased in relative abundances in the cecum of pigs fed ALP diet. At the genus level, compared to the LP diet, the ALP diet significantly increased the abundances of Lachnospiraceae UCG-005, Lachnospiraceae NK4A136 group, Phascolarctobacterium and Parabacteroides, while decreased Vibrio and Maritalea. Pigs fed the ALP diet increased Oribacterium and Lachnoclostridium when compared with the NP diet. Non-metric multidimensional scaling analysis revealed that the distribution of microbiota at each group was distinctly clustered separately along principal coordinate. In addition, quantitative PCR revealed that the ALP diet was also associated with increases in the amounts of Bacteroides, Bifidobacterium, and Lactobacillus, but a decrease in the level of Escherichia coli. Compared with the NP diet, the ALP diet enhanced the concentrations of valerate and propionate. This ALP diet also increased the concentrations of valerate and isobutyrate when compared with the LP diet. Moreover, the ALP diet was linked with a significant decline in the concentration of ammonia in the cecum. These results indicate that a LP diet supplemented with AKG can alter the balance in microbial communities, increasing the population of SCFA-producing bacteria and the amounts of Bacteroides and Bifidobacterium, while reducing the counts of Escherichia coli and the amount of ammonia in the cecum.
Introduction
The gut microbiota play a critical role in regulating and controlling of host energy metabolism (Cani and Delzenne, 2009; Yan et al., 2012). The hindgut of an animal harbors a dense and metabolically active microbiota comprising primarily bacteria that have a profound influence on immunological, nutritional, and physiological processes in the host (Su et al., 2008). Various factors can influence on the composition of the gut microbiota, such as nutritional and non-nutritional dietary components, the intestinal environment, and antibiotic use (Penders et al., 2006). In mammals, proteins and their amino acid-derived metabolites can affect the interactions between the gut microbiota and metabolism (Metges, 2000). Undigested protein in the large intestine can be fermented by indigenous bacteria to form amino acid-derived metabolites (Nyangale et al., 2015; Zhang et al., 2016), such as ammonia (NH3), branched chain fatty acids (BCFAs), hydrogen sulfide, and indolic and phenolic compounds, all of which can possibly have toxic impacts on the intestinal epithelium (Geypens et al., 1997; Luo et al., 2015). In pigs, the level of protein in the diet affect the composition and metabolism of bacteria, furthermore, the crystalline amino acids (AAs) -supplemented low-protein (LP) diet may decrease the formation of protein fermentation products, and alter microbial communities in the large intestine (Zhou et al., 2015; Zhang et al., 2016). Moreover, the addition of 3% benzoic acid to a low protein diet can reduce the NH3 concentration and NH3 emissions by 55 and 57%, respectively (Hansen et al., 2007). Benzoic acid also diminishes the growth of micro-organisms in the intestinal digesta of piglets (Kluge et al., 2006). Therefore, it is important that both researchers and swine managers understand how dietary interventions can lead to differential effects on microbial composition and fermentation.
Alpha-ketoglutarate (AKG) is a central molecule in the tricarboxylic acid cycle (Krebs cycle). It can be rapidly transaminated, by glutamate (Glu) dehydrogenase, to glutamic acid and then further aminated, by glutamine (Gln) synthetase, to form Gln (Prandini et al., 2012; Wang et al., 2016). This conversion is well-established (Wang et al., 2015):
In addition to functioning as both an energy donor and a scavenger of ammonium ions, AKG can provide a source of Gln that stimulates protein synthesis in intestinal epithelial cells (Yao et al., 2012). Furthermore, Junghans et al. (2006) reported that administration of AKG may markedly affect energy expenditure and nutrient utilization in growing pigs. Dietary supplementation with 1% AKG beneficially modulates the AMPK signaling pathway to improve energy status in the small intestine of LPS-challenged piglets (Hou et al., 2011b). Daily weight gain and feed-efficiency was improved when pigs were fed supplemented with AKG (Hou et al., 2011a; Chen et al., 2017a). In addition, the in vitro bacterial community structure is changed when AKG is introduced into diluted rumen fluid (Zhang et al., 2011). We have previously shown that dietary supplementation with AKG can potentially promote the growth of beneficial bacteria, improve intestinal microbial populations, modulate the production of short-chain fatty acids (SCFAs), and decrease the level of NH3 in the gut of growing pigs (Chen et al., 2017b).
Our study objective was to investigate how combining a LP diet with AKG might influence growth performance, cecal microbial communities, and parameters of microbial metabolism in young pigs. The data obtained here will help us further understand the mechanism through which AKG improves pig performance, and provide a scientific basis for using AKG in commercial practice.
Materials and Methods
These experiments were conducted in accordance with Chinese guidelines for animal welfare and experimental protocols, and all procedures were approved by the Committee of Animal Care at the Institute of Subtropical Agriculture, Chinese Academy of Sciences. The AKG component (purity ≥ 98%) was obtained from Hubei Yuancheng Saichuang Technology Co., Ltd., Wuhan 430064, China.
Animals, Housing, and Experimental Treatments
Twenty-seven growing pigs (Large White × Landrace, 44 ± 1 day of age) with an average initial body weight of 11.96 ± 0.18 kg were randomly assigned to one of three dietary treatments (n = 9). The pigs were housed individually in cages (1.5 m × 1.5 m). Their experimental diets were formulated, based on corn–soya bean meal, to be isoenergetic and meet the nutritional needs of these animals according to the National Research Council (NRC, 2012) (Table 1). The following dietary treatments were used: (1) NP, a normal recommended protein diet containing approximately 20% CP; (2) LP, a LP diet formulated to contain approximately 17% CP (3 percentage points below the recommended level); and (3) ALP, a LP diet supplemented with 10 g kg-1 AKG as a substitute for the regular corn components. The experiment lasted 35 days, during which time all of the pigs had ad libitum access to clean drinking water and their assigned diets.
Sample Collection and Preparation
Body weights and feed consumption were measured at the beginning and end of the 35-day experiment period. Those data were used for calculating average daily gain (ADG), average daily feed intake (ADFI), and the feed-to-gain ratio (F/G). When the feeding test ended, all of the animals were immediately anesthetized with sodium pentobarbital (50 mg/kg body weight) and killed by jugular puncture and eviscerated (He et al., 2009). Afterward, the luminal digesta of the cecum were collected and stored at -80°C before determining the concentrations of SCFAs and ammonia nitrogen (NH3–N), as well as the composition and diversity of the gut microbiota.
Analysis of Short-Chain Fatty Acids and Ammonia Nitrogen in the Cecal Digesta
The digesta of cecum were homogenized and centrifuged at 1000 × g and 4°C for 10 min. Afterward, a mixture of supernatant fluid and 25% metaphosphoric acid solution (4 mL:1 mL) was prepared for the determination of SCFAs (i.e., acetic, propionic, butyric, and valeric acids) and BCFAs (i.e., isobutyric and isovaleric acids). Analysis via gas chromatography was conducted as previously described (Kong et al., 2009).
The concentrations of NH3–N were calculated according to Nessler’s reagent colorimetric method, as described by Diao et al. (2014). Briefly, the supernatants of the cecal digesta samples were centrifuged at 5000 × g for 15 min after adding 1:10 ammonia-free water. Afterward, 1 mL of the supernatant was transferred to a 50 mL sterile tube to which 49 mL of ammonia-free water and 1 mL of potassium sodium tartrate solution were added before the mixture was briefly blended with an onavortex mixer. After 1.5 mL of Nessler’s reagent was added, the mixture stood for 10 min. Absorbance was read at 420 nm against ammonia-free water on a UV-vis Spectrophotometer (UV1100; MAPADA, Shanghai, China). Based on the absorbance of the standard, we calculated the NH3–N concentrations as follows: NH3–N (N, mg/L) = m × 1000/V, where m represented the NH3–N concentration corrected by the standard curve and V represented the volume of the supernatant.
Bacterial Quantification by Real-Time PCR
Total bacterial DNAs were extracted from the contents of each intestinal sample (0.2 g) according to a previously described protocol (Kraler et al., 2016), using a commercially available QIAamp DNA Stool Mini Kit (Qiagen, Hilden, Germany). Those extracts were stored at -80°C. They were then quantified on a Nanodrop 2000 Spectrophotometer (Thermo Scientific, Courtaboeuf, France) before the results were adjusted to a concentration of 10 ng μL-1.
Methods based on 16S rRNA were used to assess the abundances of total bacteria, Bacteroides, Bifidobacterium, Firmicutes, Escherichia coli, Lactobacillus, Clostridium coccoides, and the Clostridium leptum subgroup, as described previously (Kong et al., 2014; Fleury et al., 2016). All PCR primers used in this study are listed in Table 2. Duplicate sample analysis was performed in a mixture (final volume, 10 μL) that contained 1 μL of diluted DNA sample, 0.2 μM of each primer, and 0.25 μM of TaqMan probe, using a 1× of SYBR Premix Ex Taq II kit (TaKaRa Bio Inc., Shiga, Japan). The qPCR protocol for assaying E. coli included 0.3 μM of each primer and 0.1 μM of probe, while those reactions for Firmicutes, Clostridium coccoides, and the Clostridium leptum subgroup used a concentration of 0.4 μM of each primer. The amplification program entailed 95°C for 30 s; followed by 40 cycles of 95°C for 5 s and 60°C for 30 s; and then a final melting-curve for SYBR Green tests. The melting curve analysis and size-determination of amplificates on agarose gels verified that the target fragments had been amplified. Standard curves were generated as described by Qi et al. (2011). For each sample and each bacterial group, results were expressed in log10 copies of 16S rRNA genes per g of intestinal content material (Metzlerzebeli et al., 2015).
DNA Extraction, PCR Amplification, Library Preparation and Sequencing
Fifteen growing pigs from the three treatment groups (5 per group) were randomly chosen for 16S rRNA sequencing analyses. Total genomic DNA was isolated from the samples of cecal digesta by using QIAamp DNA Stool Mini Kits according to the manufacturer’s instructions. The concentration of the extracted DNA was determined with the NanoDrop-1000 Spectrophotometer (NanoDrop Technologies Inc., Wilmington, DE, United States), and was stored at -80°C before further analysis.
To analyze the taxonomic composition of the bacterial community, we used 16S rRNA gene amplicons to determine the diversity and make structure comparisons of the bacterial communities in each of these samples. Sequencing was performed at Novogene Bioinformatics Technology Co. Ltd., Beijing, China. The PCR amplifications were conducted with the barcoded primer pair 341f/806r set, which amplifies the V3–V4 fragments of the 16S rDNA gene (341F:CCTAYGGGRBGCASCAG, 806R: GGACTACNNGGGTATCTAAT) (Muyzer et al., 1993; Caporaso et al., 2011). PCR reactions were performed in a volume of 30 μL containing 12 μL sterile water, 1.0 μL DNA template, 1.0 μL of each primer, and 15 μL 2 × Phusion Master Mix (New England Biolabs, United States). The PCR cycle conditions were as follows: initial denaturation at 98°C for 1 min, followed by 30 cycles at 98°C for 10 s, 50°C for 30 s, and 72°C for 30 s, and a final extension step at 72°C for 5 min. Resulting amplicons were confirmed on 2% agarose gels containing ethidium bromide.
All amplicons were in the size range of 400–450 bp, and were purified using a GeneJET Gel Extraction Kit (Thermo Fisher Scientific, Carlsbad, CA, United States). Following quantitation, equal concentrations of the purified amplicons were combined into a single tube. Sequencing libraries were generated using a NEB Next Ultra DNA Library Prep Kit for Illumina (New England Biolabs, Ipswich, MA, United States) following manufacturer’s recommendations, and index codes were added. The library quality was assessed on a Qubit@ 2.0 Fluorometer (Thermo Fisher Scientific, Carlsbad, CA, United States) and Agilent Bioanalyzer 2100 system. Sequencing was conducted on an Illumina MiSeq2500 platform, which generated 250-bp paired-end reads.
Bioinformatics Analysis
Raw data were screened and assembled by FLASH v1.2.71 (Magoč and Salzberg, 2011) and QIIME (V1.7.0)2 (Caporaso et al., 2010) software packages. Chimera sequences were removed through the UCHIME algorithm (UCHIME Algorithm3) (Edgar et al., 2011) to form the “effective sequences” collection for each sample. Uparse software (Uparse v7.0.1001)4 (Edgar, 2013) were used for analysis of the sequences and determination of the Operational Taxonomic Units (OTUs) with an identity threshold of 97%. Meanwhile, we picked a representative sequence for each OTUs and used the RDP classified to assign taxonomic data to each representative sequence. Taxonomy classifications were assigned against RDP Classifier (Version 2.2)5 (Wang et al., 2007) and the Greengenes database6 (DeSantis et al., 2006). The taxon abundance of each sample was then determined at the levels of phylum, class, order, family, and genera. All of the analyses from clustering to alpha diversity (Chao1, ACE, Simpson and Shannon) were calculated by QIIME software (Version 1.7.0) and displayed with R software (Version 2.15.3). Non-metric Multidimensional Scaling (NMDS) plots based on Bray–Curtis distance was performed to show the group differences in microbial community structure. Statistical testing among variation in microbial community composition was carried out using the analysis of similarity (ANOSIM). Hierarchical clustering of samples was completed using UPGMA. All the raw sequence data in the present study have been deposited in the sequence read archive (SRA) at NCBI under the accession number SRP125897.
Statistical Analysis
Growth performance indices were assessed by analysis of variance (ANOVA). A non-parametric ANOVA (Kruskal–Wallis test) was performed to analyze cecal SCFAs, ammonia concentrations and bacterial populations by qPCR. Differences in alpha diversity and relative abundances of bacterial phyla between samples were analyzed by the Mann–Whitney U-test. All analyses were performed using IBM SPSS v. 20.0 (SPSS Inc., Chicago, IL, United States). For all tests, values of P < 0.05 were considered statistically significant differences. The abundance of genus with significant differences between groups were assessed by analysis of MetaStat, significant correlations were found (q < 0.05), extremely significant differences was considered at q < 0.01.
Results
Growth Performance
The effects of LP diets supplemented with AKG on feed intake and growth performance are shown in Figure 1. Among the diet groups, no significant differences were found in ADG (P = 0.099), ADFI (P = 0.282), or F/G (P = 0.326). However, pigs fed the ALP diet showed a 7.23% increase in average daily gain when compared with those in the LP group (P = 0.148).
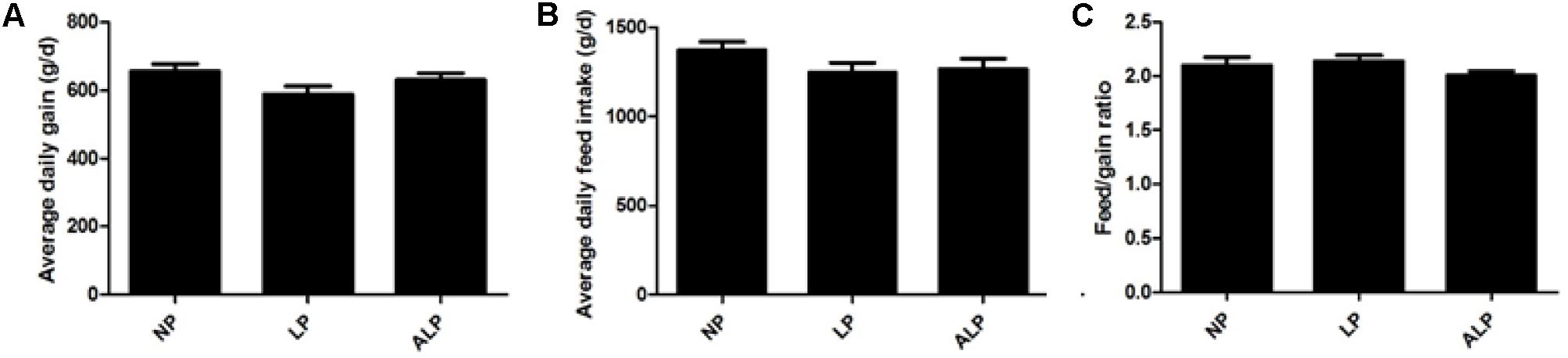
FIGURE 1. The growth performance of growing pigs fed various experimental diets. (A) Average daily gain of LP-diet-fed growing pigs with AKG supplementation. (B) Average daily feed intake of LP- diet-fed growing pigs with AKG supplementation. (C) Feed intake/gain of LP-diet-fed growing pigs with AKG supplementation. ADG, average daily gain; ADFI, average daily feed intake; F/G, feed intake/gain. NP, normal protein; LP, low protein; ALP, AKG plus low protein. Data are presented as the means ± SEM (n = 9). Differences were assessed by ANOVA.
DNA Sequence Coverage and Microbial Community Structure in Cecal Digesta
To evaluate the impact of a LP diet supplemented with AKG on microbial communities, a total number of 1,055,010 of V3–V4 16S rRNA valid sequences reads were obtained from 15 samples (70,334 ± 1,000 per sample), including an average of 66,241 (NP group), 72,001 (LP), and 72,760 (ALP) raw reads in the current study. After trimming, assembly, and quality filtering, an average of 35,384, 39,546, and 41,270 sequences were selected for further analysis from the NP, LP, and ALP groups, respectively. When all of the samples were considered, the sequence reads numbered 32,694–45,537 per sample, with an average of 38,384 (Supplementary Table S1). A total of 30,000 effective sequences was extracted from each sample for comparisons at the same sequencing depth.
A total of 8868 OTUs were obtained after filtering out reads with low quality segments, with an average of 591 ± 14 OTUs per sample, and from the Venn analysis of OTUs (Figures 2A–C), 11, 48, and 24 unique OTUs were identified in NP, LP, and ALP groups, respectively(Supplementary Table S1 and Figure 2D). At a genetic distance of 3%, no remarkable differences were found in the diversity indices (Shannon and Simpson) and richness estimators (ACE and Chao1) of the cecal microbiota among treatment groups (P > 0.05) (Supplementary Figure S1 and Supplementary Table S2). At the phylum level, five phyla – Bacteroidetes, Cyanobacteria, Firmicutes, Proteobacteria, and Spirochaetes – had a relative abundance greater than 0.5% in at least one experimental group. Of these, Firmicutes predominated in all samples, with a relative abundance of 41.69–56.57%, followed by Bacteroidetes (32.25–35.12%), Proteobacteria (7.11–14.04%), Cyanobacteria (0.58–4.78%) and Spirochaetes (0.41–2.83%). When the LP diet was supplemented with AKG, widespread changes were induced in the gut microbial community structure at the phylum level when compared with the LP diet alone. In particular, abundances were increased for Firmicutes (48.23% vs. 41.69%) (P = 0.310) but decreased for Cyanobacteria (0.06% vs. 4.78%) (P = 0.421) and Spirochaetes (1.80% vs. 2.83%) (P = 0.690). In contrast, both Proteobacteria (14.04% vs.7.11%) (P = 0.841) and Spirochaetes (1.80% vs.0.41%) (P = 0.095) were increased in the ALP group when compared with the NP group (Figure 3). The proportion of some phyla (Euryarchaeota, Tenericutes, Actinobacteria, Elusimicrobia, and Verrucomicrobia) was less than 1% of total microbial community. Within the bacterial population, the top 35 genera were identified across all samples (Figure 4). The abundance of genus Lachnospiraceae UCG-005(11.76% vs. 0.42%) (q = 0.041), Lachnospiraceae NK4A136 group (0.58% vs. 0.07%) (q = 0.042) in the Lachnospiraceae family, Phascolarctobacterium (0.71% vs. 0.19%) (q = 0.033) in the Acidaminococcaceae family and Parabacteroides (0.23% vs. 0.06%) (q = 0.012) in the Porphyromonadaceae family were increased in the ALP group when compared with the LP group, while Vibrio (0.03% vs. 0.24%) (q = 0.032) in the Vibrionaceae family and Maritalea (0.00067% vs. 0.01%) (q < 0.01) in the Hyphomicrobiaceae family were decreased. Supplementing the LP diet with AKG caused the abundances of Oribacterium (0.57% vs. 0.29%) (q = 0.021) and Lachnoclostridium (0.93% vs.0.44%) (q < 0.01) in the Lachnospiraceae family to increase when compared with the NP group (Figure 5). Furthermore, the composition of Oribacterium, Lachnospiraceae and Phascolarctobacterium in cecal samples based on bacterial quantification by real-time PCR (Supplementary Material) was represented in Supplementary Figure S2. The amounts of Lachnospiraceae and Phascolarctobacterium were significantly increased in pigs receiving the ALP diets when compared with the LP group (P = 0.027). Supplementing the LP diet with AKG caused the abundances of Oribacterium (P = 0.042) to increase when compared with the NP group.
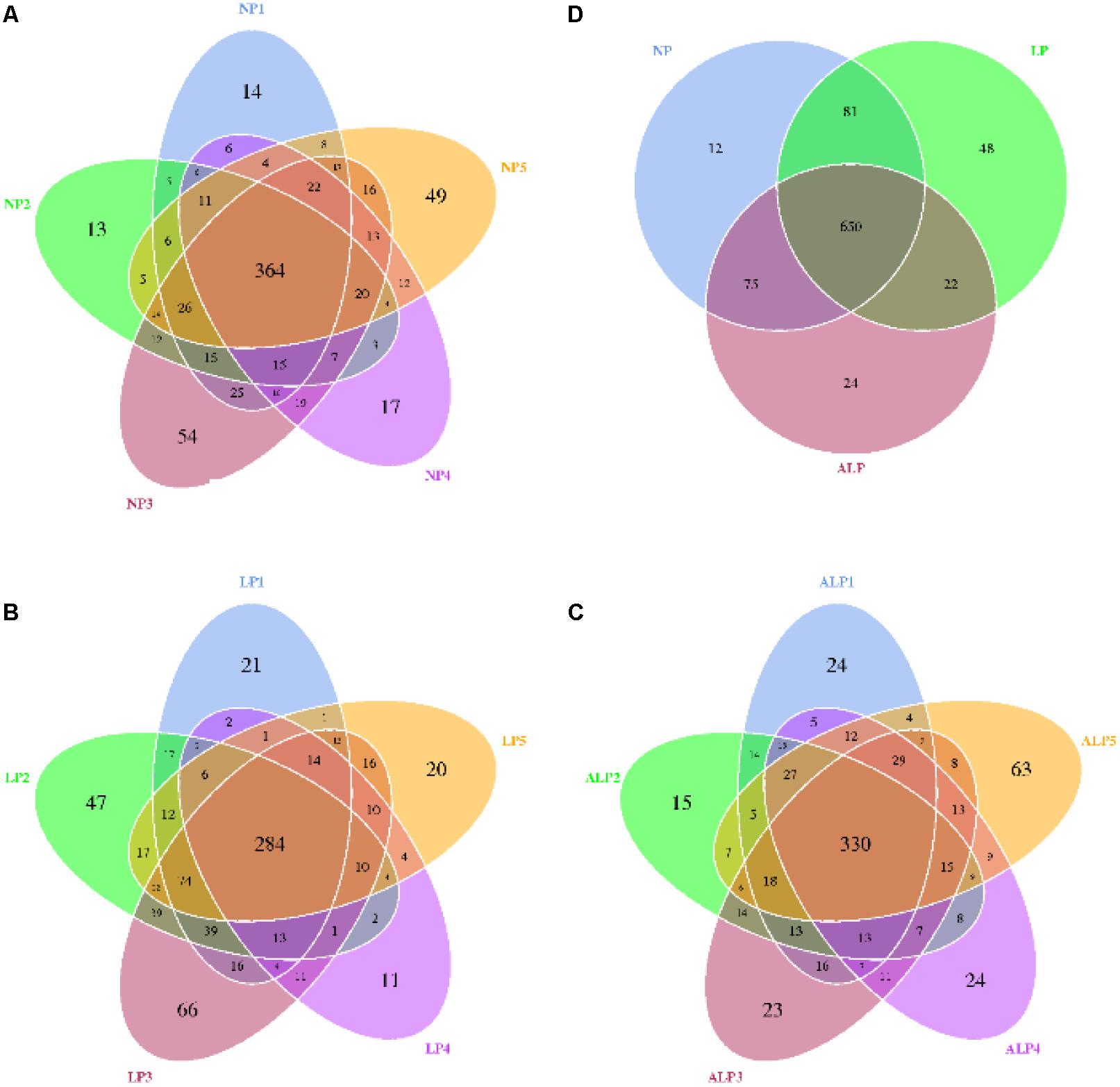
FIGURE 2. Shared OTUs analysis of the different samples. Venn diagram showing the unique and shared OTUs in the different samples. NP, LP, and ALP are samples mentioned in Supplementary Table S1. (A) for NP1, NP 2, NP 3, NP 4, and NP 5 samples; (B) for LP1, LP 2, LP 3, LP 4, and LP 5 samples; (C) for ALP1, ALP 2, ALP 3, ALP 4, and ALP 5 samples; (D) for NP, LP, and ALP samples; NP, normal protein; LP, low protein; ALP, AKG plus low protein.
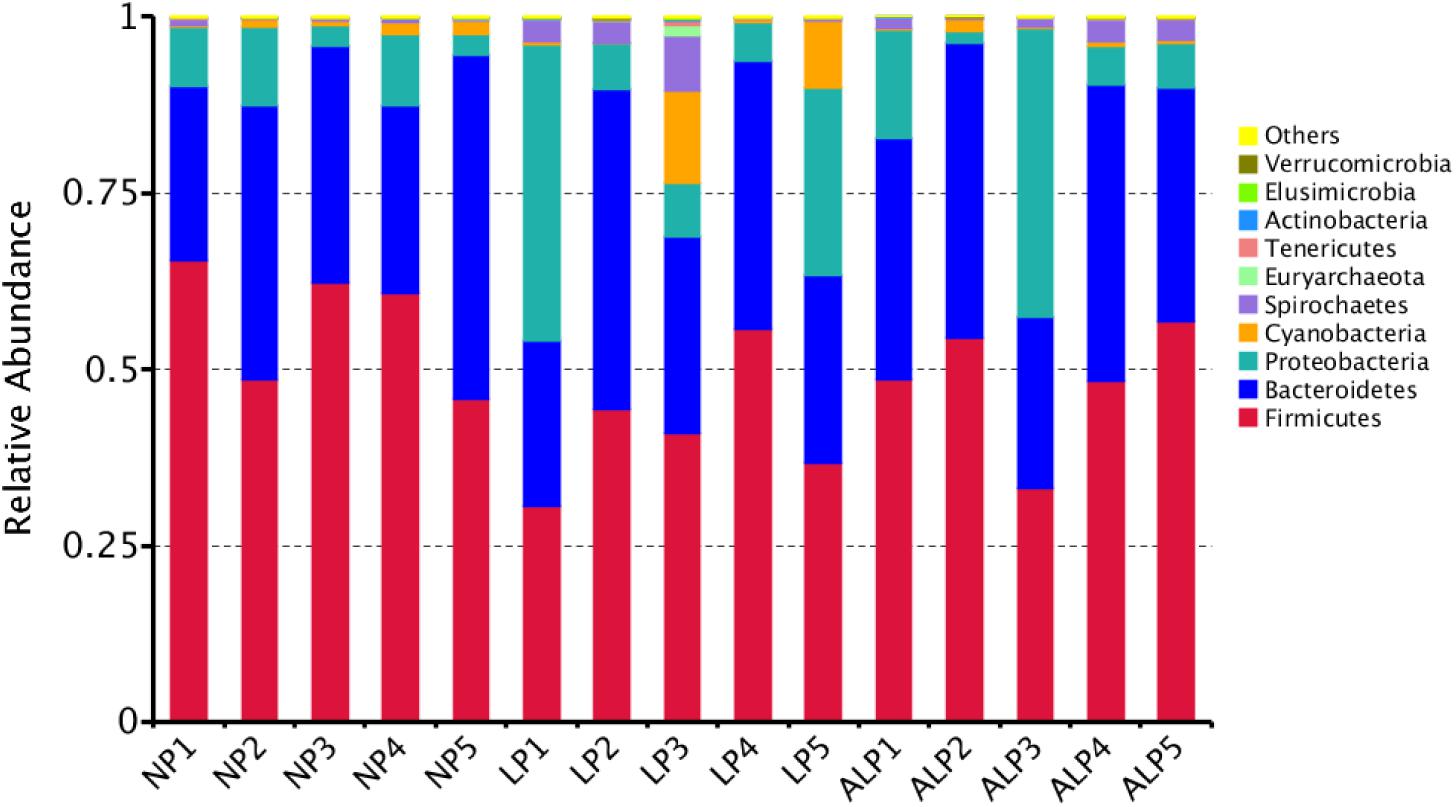
FIGURE 3. Relative abundance of cecal bacteria, at phylum level, in pigs fed different diets. Representative sequences for each OTU were used to annotate taxonomic information. NP, normal protein; LP, low protein; ALP, AKG plus low protein.
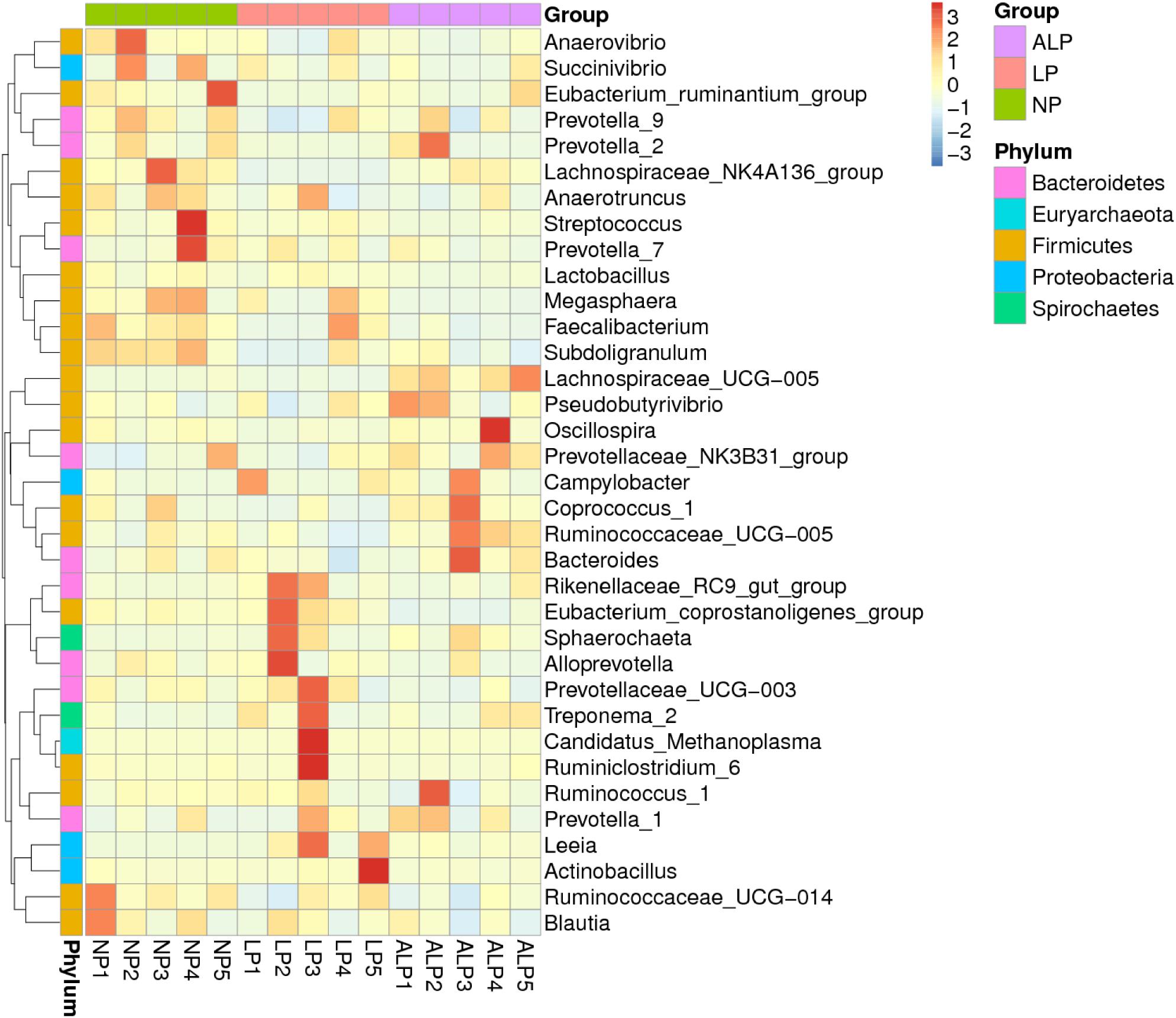
FIGURE 4. Heatmap based on hierarchical clustering solution (Bray–Curtis distance metric and complete clustering method) for 15 pigs fed three different diets, shown across columns. Rows represent top 35 microbial genera. NP, normal protein; LP, low protein; ALP, AKG plus low protein.
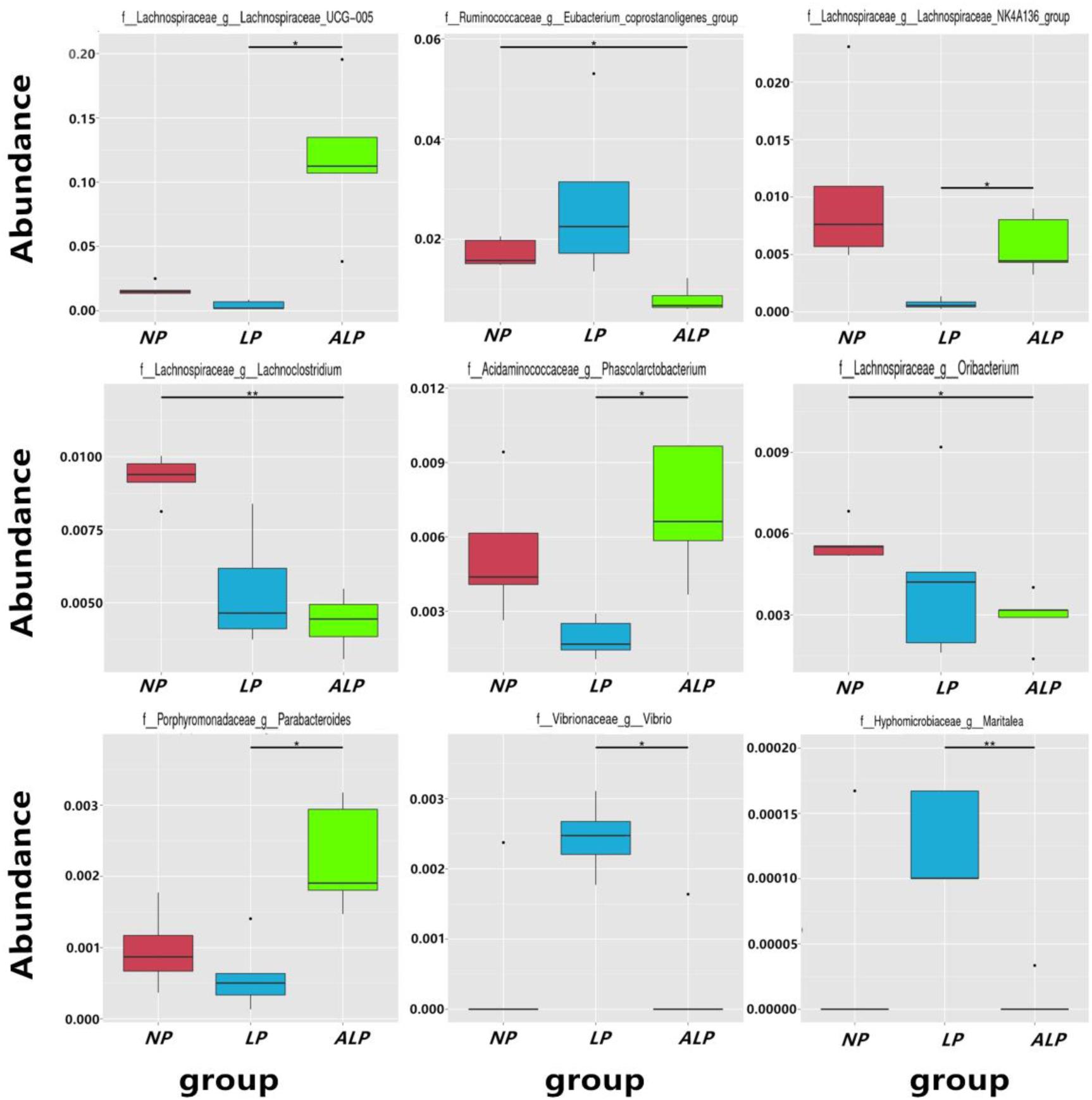
FIGURE 5. Relative abundance of microbial genera (percentage) in pig cecum that were significantly affected by dietary treatment. NP, normal protein; LP, low protein; ALP, AKG plus low protein. ∗Indicates significant difference between the two groups (q value < 0.05), ∗∗indicates the difference between the two groups was significant (q value < 0.01).
The relationships in the cecal bacterial communities between samples were compared by NMDS and ANOSIM based on Bray–Curtis (Singh et al., 2015). The NMDS plot indicated that the distribution of microbiota at each group was distinctly clustered separately along principal coordinate (Figure 6A). ANOSIM revealed significant differences in the structure of gut microbiota among different groups (Figure 6A; ALP-NP r = 0.648, p = 0.004; LP-NP r = 0.168, p = 0.043; ALP-LP r = 0.204, p = 0.078), indicating that ALP diet could exert a notable effect on the cecal microbial community of piglets. Furthermore, the HCA indicated that the similarity dendrogram was separated by AKG rather than protein level (Figure 6B).
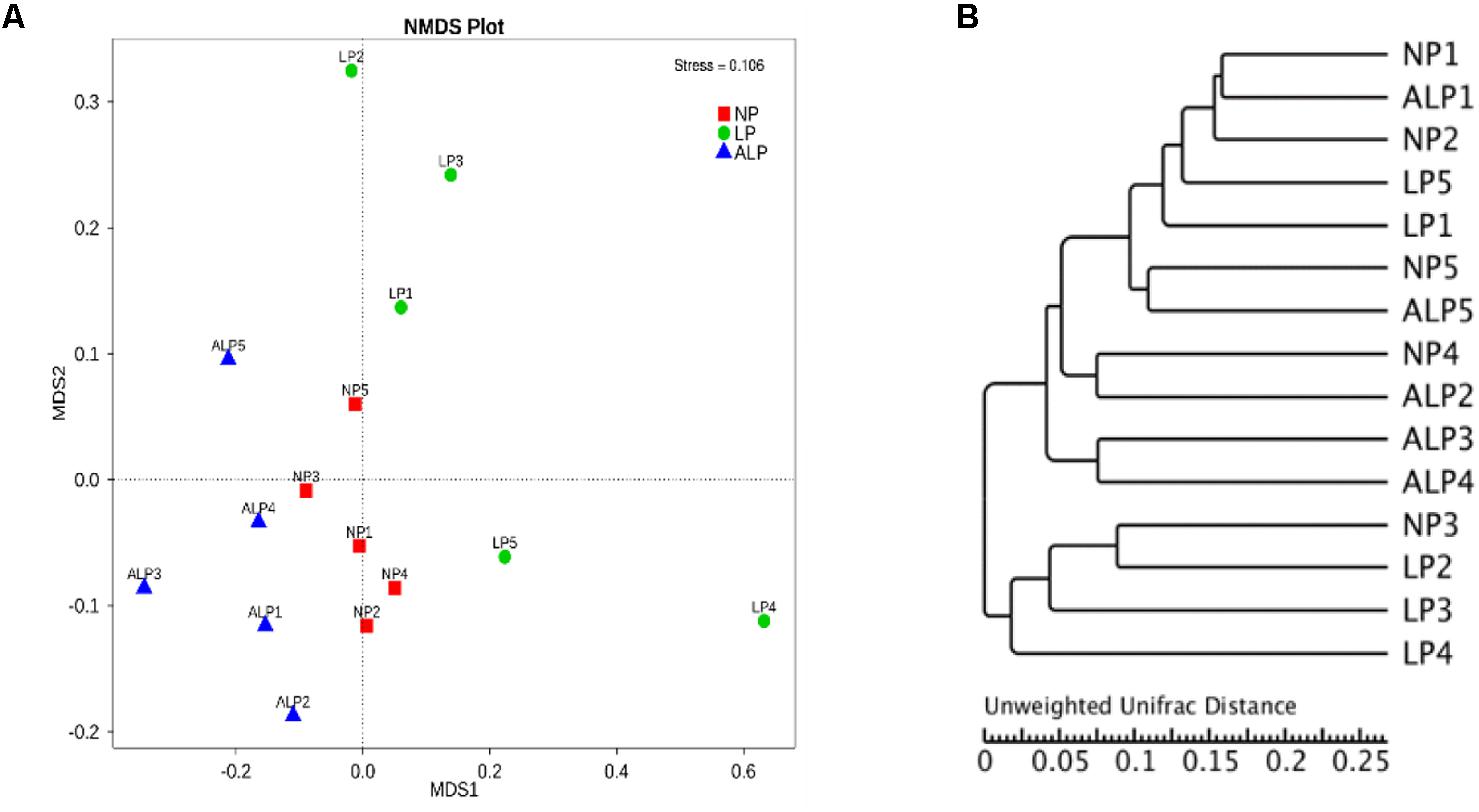
FIGURE 6. Comparison of cecal microbial community structures within growing pigs fed different diets. (A) Non-metric multidimensional scaling (NMDS) based on weighted UniFrac distance among samples of different treatments, based on stress < 0.2 with significantly different abundance among groups (NP, LP, and ALP), (B) UPGMA tree, all revealing significant differences among treatments based on Unweighted UniFrac distances of OTU community. NP, normal protein; LP, low protein; ALP, AKG plus low protein.
Quantitative Analysis of the Intestinal Microbiota
To examine the quantitative changes to intestinal microbiota in the cecum, we performed real-time PCR for the total bacteria population as well for some major bacterial groups. Figure 7 shows that the amounts of Bacteroides were significantly increased in pigs receiving the ALP and LP diets when compared with levels in pigs fed the NP diet (P = 0.032). Moreover, the amounts of Bifidobacterium significantly increased (P = 0.013), Escherichia coli significantly decreased (P = 0.025), and the population of Lactobacillus slightly increased (P = 0.081) in the ALP group when compared with the NP and LP groups. However, no significant differences among treatment groups (P > 0.100) were found for any of the other examined populations in the cecum.
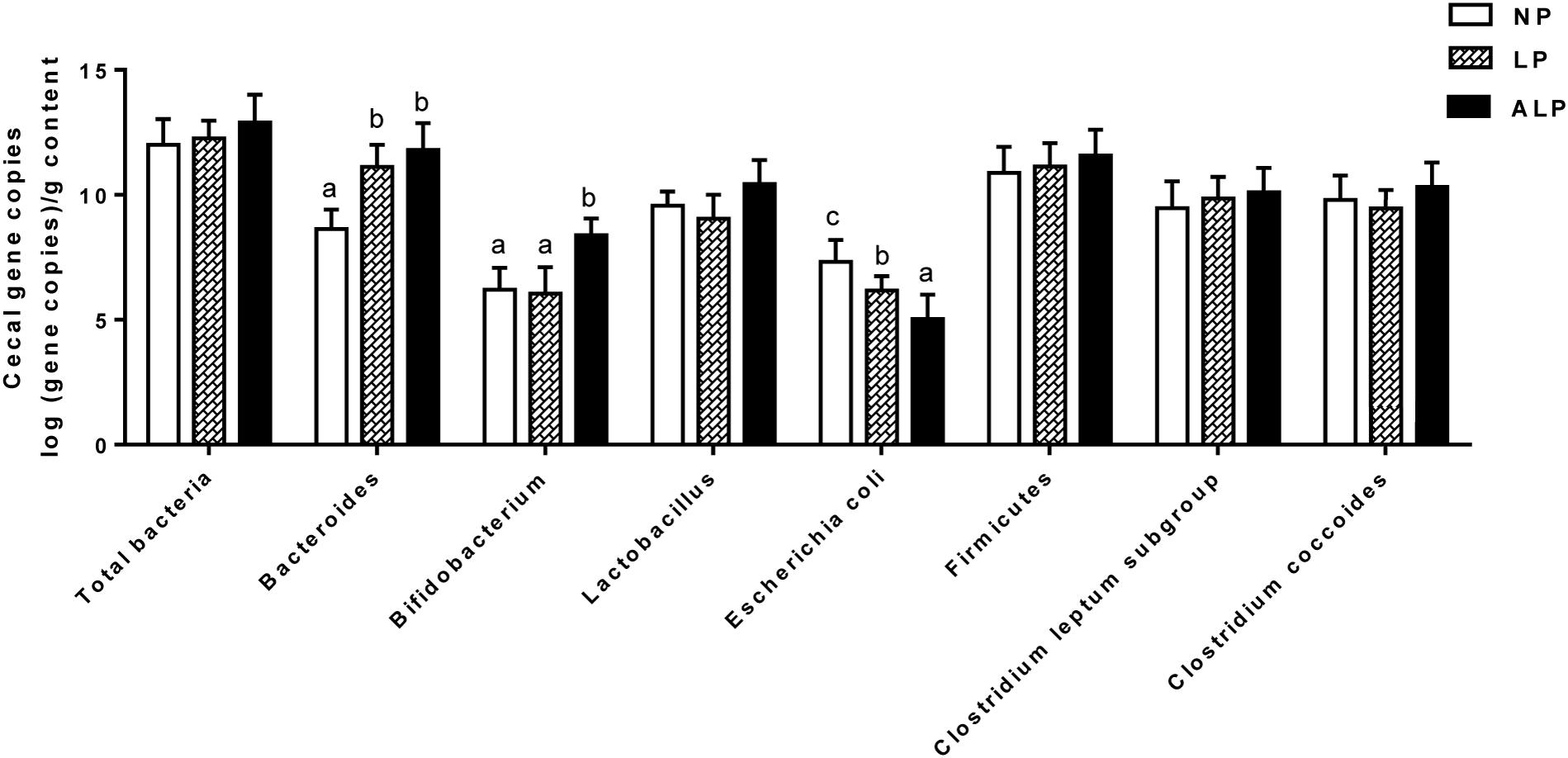
FIGURE 7. Comparison of major bacterial taxonomic populations in cecum among dietary treatments, using real-time PCR analysis. NP, normal protein; LP, low protein; ALP, AKG plus low protein. For each bacterial group, bars not labeled with same letters indicate values are significantly different at P < 0.05.
Fermentation Metabolites of Cecal Digesta
The concentrations of SCFAs and ammonia in cecal digesta are shown in Figure 8. While the concentrations of acetate, butyrate, isobutyrate, and total SCFAs did not differ significantly among groups, the isobutyrate concentration was significantly decreased (P = 0.021) in response to the LP diet. The valerate concentration was significantly decreased (P = 0.019) due to the LP diet when compared with the effect of the ALP diet, while the levels of propionate were significantly increased in animals fed with either the LP or ALP diets (P = 0.038) when compared with the NP group.
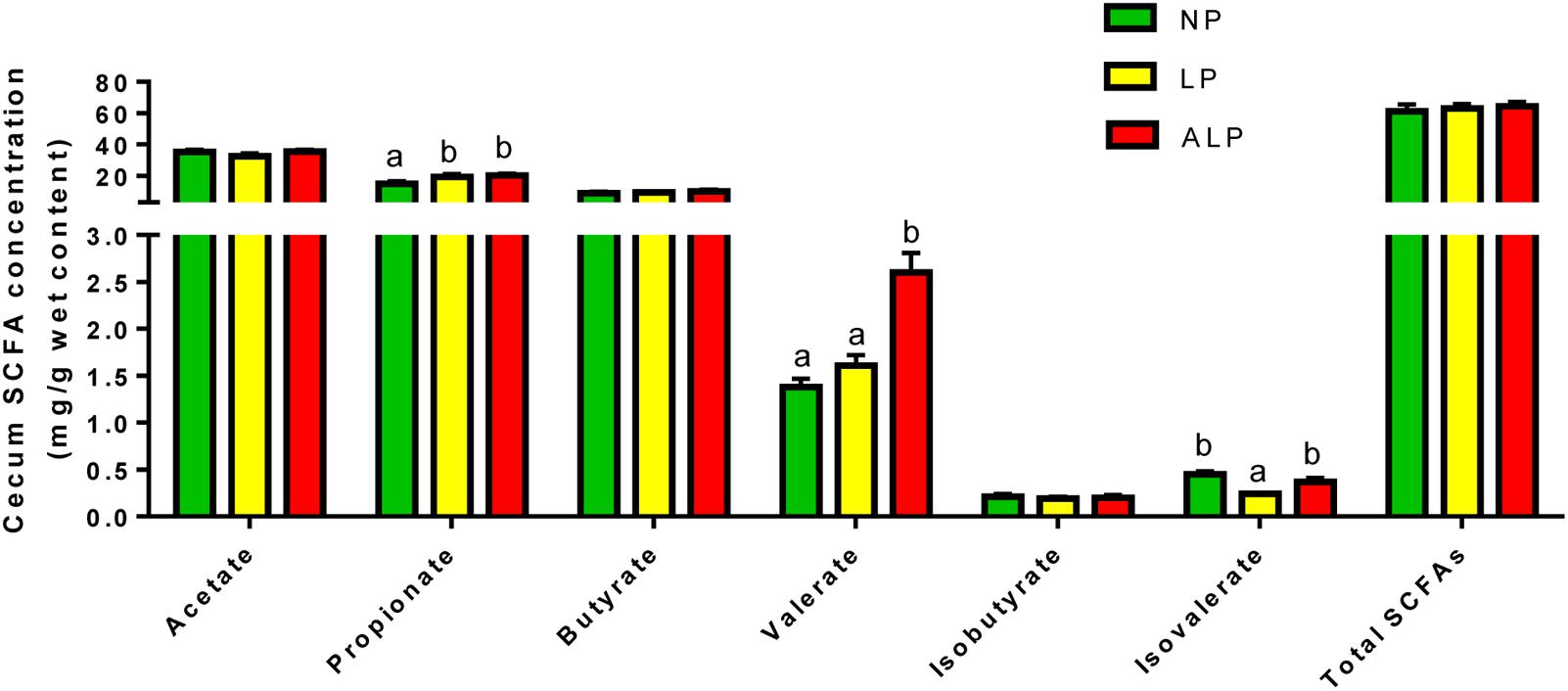
FIGURE 8. Comparison of cecal short chain fatty acids (SCFAs) (mg/g) among dietary treatments. NP, normal protein; LP, low protein; ALP, AKG plus low protein. For each group of acids, bars not labeled with same letters indicate values are significantly different at P < 0.05.
Ammonia is produced through bacterial deamination of amino acids. Compared with the NP diet, the LP and ALP diets were linked with significant reductions (P = 0.002) in the ammonia concentration in the cecum (Figure 9).
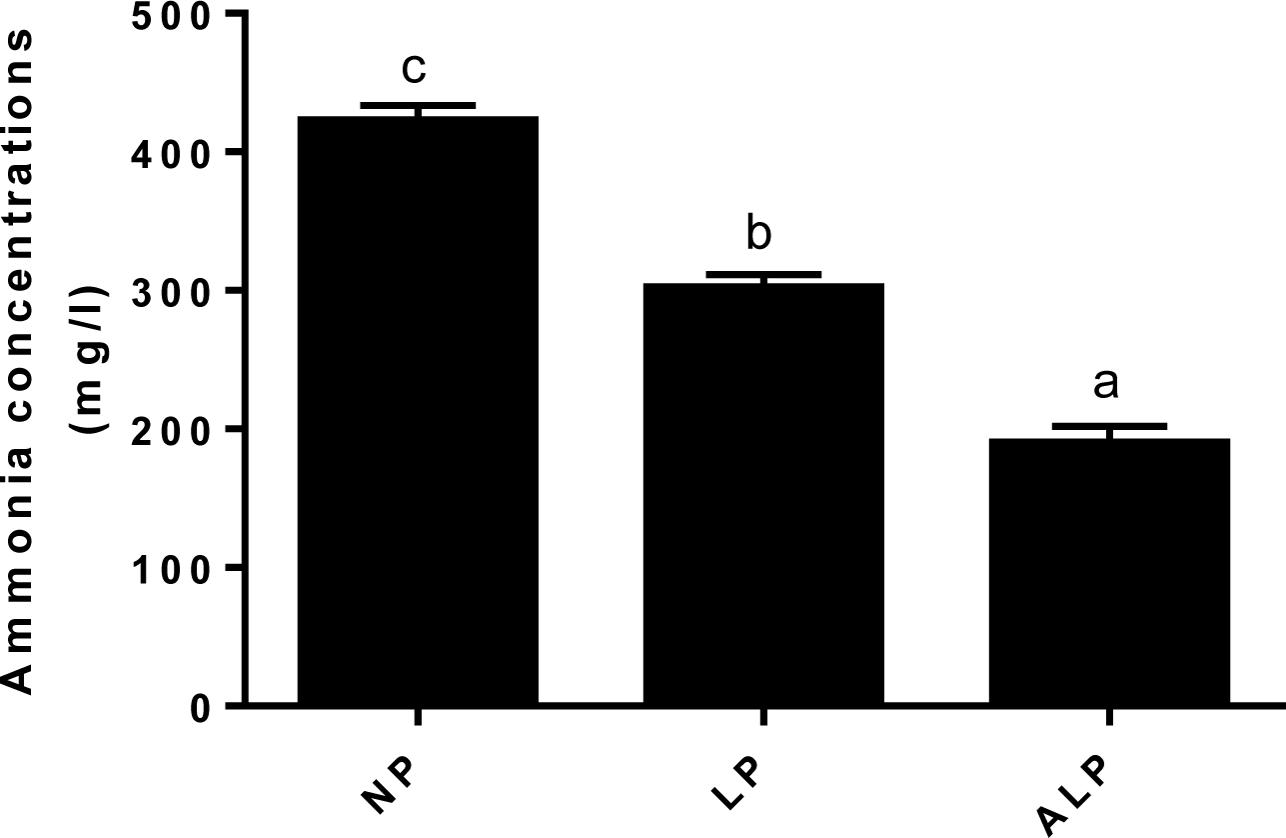
FIGURE 9. Comparison of ammonia nitrogen (mg/L) concentrations among dietary treatments. NP, normal protein; LP, low protein; ALP, AKG plus low protein. Bars not labeled with same letters indicate values are significantly different at P < 0.05.
Discussion
Organic acids and their salts are considered a class of natural growth promoters that have long been used as feed additives in pig nutrition programs to reduce the buffering capacity of starter diets (Giannenas et al., 2016). Various organic acids (e.g., formic, citric, fumaric, malic, and benzoic) can improve growth performance and regulate the intestinal microbiota in pigs (Partanen and Mroz, 1999; Piva et al., 2002; Kluge et al., 2006; Øverland et al., 2008; Halas et al., 2010). However, the strategy of using a low protein diet balanced with AAs has no influence on growth performance in commercial swine operations (Opapeju et al., 2008) and broiler chickens (Kobayashi et al., 2013). Our research goal was to examine how, while applying current production practices, a low protein diet (3% below normal) could be supplemented with AKG to improve growth performance, the microbial communities, and metabolic profiles in the cecum of a growing pig. In fact, the LP diet alone had no impact on the values calculated for ADG, ADFI, or F/G. This outcome is consistent with that reported by Le Bellego and Noblet (2002), who showed that reducing the protein level from 204 to 169 g/kg did not affect feed intake nor daily weight gain of the piglets (12 kg initial BW). However, we did note here that the ADG was increased by 7.23% in pigs that received an LP diet supplemented with AKG when compared with the results from the LP group. This is in accord with findings from previous studies (Hou et al., 2011a; Chen et al., 2017a). It is possible that the dietary AKG supplementation promotes intestinal development (Hou et al., 2010), and increases AA- and energy-digestibility and nitrogen deposition (Chen et al., 2017a), while reducing the populations of harmful bacteria and modifying their metabolites within the gastrointestinal tract (Chen et al., 2017b). Furthermore, AKG is an intermediate of the citric acid cycle and the natural ubiquitous collector of amino groups in body tissues. It has been reported that AKG can stimulates AMPK phosphorylation and oxidation of energy substrates in the intestinal mucosa (Hou et al., 2011b), thereby inactivating one of its downstream target enzymes, acetyl-CoA carboxylase (ACC) (Dyck et al., 1999), which converts acetyl-CoA into malonyl-CoA (an inhibitor of fatty acid oxidation and a substrate for fatty acid synthesis) (Hou et al., 2011b) and enhancing ATP supply, thereby improving fat storage and weight gain. This was reflected by the rise in ADG in those pigs fed ALP diets over the entire experimental period.
Because the gut microbial community has potentially important roles in swine growth performance and health, its composition and metabolic activities in various nutritional and physiological contexts deserve close attention (Hill et al., 2005). It has been demonstrated that the composition of the intestinal and fecal microbiota (including the bacterial profiles of the mucosa and digesta) changed in pigs from birth to the finishing phases (Konstantinov et al., 2006; Kim et al., 2011). We comprehensively evaluated the diversity in that microbial community as well as the composition of cecal samples from growing pigs by using 16S rRNA gene sequencing. On average, 38,384 effective reads were obtained for each sample (Supplementary Table S1), with high coverage (>99.07%) (Supplementary Table S2). Furthermore, the microbial richness and diversity indices in the cecum were not influenced by diet (Supplementary Figure S1 and Supplementary Table S2). At the phylum level, the cecal microbial communities in growing pigs were dominated by Firmicutes (41.69–56.57% of the total microbial content), Bacteroidetes (32.25–35.12%), and Proteobacteria (7.11–14.04%) (Figure 3). This observation is in accordance with the findings of Ji et al. (2017) and Isaacson and Kim (2012). We also found that, at the genus level, the AKG-supplemented LP diet increased the abundances of Lachnospiraceae, and Phascolarctobacterium species from the Firmicutes phylum, and Parabacteroides species from the Bacteroidetes phylum (Figures 4, 5). Some bacteria belonging to the Firmicutes phylum can efficiently ferment fiber into butyrate, while some bacteria from the Bacteroidetes phylum ferment fiber into acetate and propionates (Zhou et al., 2017). Moreover, microbial community structure in piglets fed with ALP was remarkably different from that in LP group or NP (Figure 6A). Furthermore, the HCA showed that the similarity dendrogram was separated by AKG rather than protein level (Figure 6B). This suggests that the change in gut microbiota structure results from its accommodation to an intestinal environment created by adding AKG to the diet. As described below, there were some changes in intestinal microbial composition, and consequently altered metabolites types and concentrations of cecal digesta under ALP diet.
Bacteroides and Bifidobacterium are the two main microbial genera that most significantly affect energy homeostasis and substrate fermentation in the hind gut (Tennyson and Friedman, 2008). In pigs, Bacteroides species may compose a small fraction of the phylum Bacteroidetes community while other members of this phylum may bound up with the development of obesity (Yan et al., 2012). We found that the LP diet increased the amounts of cecal Bacteroides (Figure 7), an outcome consistent with that from a previous study (Zhang et al., 2016). The ALP diet also increased the amounts of cecal Bacteroides and Bifidobacterium (Figure 7), perhaps leading to AKG-induced fat accumulations. This was reflected by the rise in ADG in those pigs fed ALP diets over the entire experimental period. These findings partly agree with those described previously. For example, Chen et al. (2017b) demonstrated that supplementing the dietary feed with AKG elevated the cecal Bifidobacterium population in growing pigs. Our investigation also revealed that a LP diet supplemented with AKG increased the level of cecal Lactobacillus and decreased the E. coli population when compared with the other diet groups. These microorganisms – Lactobacillus and E. coli – are generally considered to be beneficial and harmful, respectively, to the host animal, and Lactobacillus is a commonly used probiotic and is thought to create a barrier against pathogen colonization (e.g., by coliform bacteria) in the proximal part of the alimentary tract of young animals (Knarreborg et al., 2002). Because AKG is a weak acid (Filip and Pierzynowski, 2007; Liem et al., 2008), it can only diminish the effect of intestinal pathogenic bacteria (such as coliforms), but not effectively inhibit the activity of useful bacteria, including Lactobacillus, largely because of the pH-insusceptibility of that organism (Kazempour and Jahanian, 2017). Furthermore, Glu and several other amino acids are primarily utilized by the bacteria in the intestines of pigs, where they play crucial roles in preserving intestinal functions and health, including the balance of microflora (Dai et al., 2010, 2012).
Profiles of microbial metabolites can be used to represent microbial activity and intestinal health. Some beneficial bacteria (e.g., Bacteroides and Bifidobacterium) can ferment carbohydrates to produce high concentrations of SCFAs (e.g., acetic acid, butyric acid, valeric acid, and propionic acid), which in turn can decrease the pH of their environment and inhibit the growth of other bacteria (Arunachalam, 1999; Olukosi and Dono, 2014; Chen et al., 2017b). Generally, valeric acids and branched-chain (isobutyric and isovaleric acids) are considered as the end products of protein fermentation (Williams et al., 2015). Protein levels in dietary feed can affect microbial fermentation in the cecum (Zhang et al., 2016). When compared with the NP diet, the LP diet can decrease the amounts of isobutyrate and isovalerate in the cecum (Zhou et al., 2015). Similarly, we found that the LP diet decreased the isobutyrate concentration (Figure 8). Furthermore, supplementing this LP diet with AKG increased the concentrations of valerate and propionate (Figure 8). We have previously reported that the dietary AKG supplementation enhances the concentrations of butyric acid and valeric acid in the cecal digesta (Chen et al., 2017b). Halas et al. (2010) also showed that dietary benzoic acid supplementation increases the relative amount of valeric acid in the cecum. In the present study, it’s possible that the increase of valerate and propionate could be well explained by the increase of Bacteroides and Bifidobacterium (Arunachalam, 1999). All of these results suggest that fewer indigestible dietary proteins are entering the cecum and are being degraded by cecal microbial proteases. Energy is the limiting factor for microbial growth in the hindgut, the primary site of intestinal fermentation (Piva et al., 2002). Therefore, AKG can serves as a metabolic fuel for the gut in animals fed an LP diet, thus sparing other oxidative fuels (Lambert et al., 2002). As a valuable source of energy for the intestinal microbiota, AKG improves bacterial fermentation in those parts of the digestive tract, thereby modulating the production of SCFAs and BCFAs.
Ammonia is a toxic catabolite produced through urea hydrolysis and the degradation of microbial amino acids, may interfere with epithelial cell turnover and depress the growth performance of pigs (Walsh et al., 2007). Swine productivity is not necessarily diminished if one can reduce the amount of crude protein and, ultimately, that of ammonia while still maintaining an adequate level of essential amino acids (Canh et al., 1998; Hayes et al., 2004; Hansen et al., 2007). Similarly, we found here that pigs receiving the LP diet showed a decreased ammonia concentration in the cecum (Figure 9). That concentration was also significantly reduced in the cecum when the LP diet was supplemented with AKG. These results are in accord with those reported previously (Hansen et al., 2007; Chen et al., 2017b). The LP diet supplementation with crystalline AAs may deter the formation of protein fermentation products in the large intestine (Luo et al., 2015; Zhou et al., 2015). Moreover, the addition of appropriate exogenous organic acids to pig diets may increase the apparent digestibilities of protein and AAs, while also reducing the production of NH3 (Partanen and Mroz, 1999; Murphy et al., 2013). All of these findings suggest that deamination and decarboxylation of dietary and/or endogenous protein by microorganisms in the gastrointestinal tract are altered by such supplementation and respond to an LP diet. These responses could be due to a decline in the microbial fermentation. LP diet may decrease amino acid breakdown by cecum bacteria or increased incorporation of amino acids into microbial biomass (Pieper et al., 2012; Zhang et al., 2016). The improvement on amino acids digestion and absorption results in better N digestibility and less NH3 excretion (Chen et al., 2017a). As an intermediate of the citric acid cycle and a natural, ubiquitous collector of amino groups in body tissues, AKG can decrease the level of free NH3 by reducing glutamate and glutamine oxidation and/or by incorporating NH3 into glutamate by the reversible enzyme GDH (Wirén et al., 2002; Lambert et al., 2006). In addition, dietary AKG supplementation can increase glutamine synthetase gene expression in intestine of common carp (Cyprinus carpio) (Dong et al., 2014). Therefore, this supplement has a beneficial effect on N metabolism (Wirén et al., 2002) and can decrease the toxicity of ammonium ions in an organism (Stoll et al., 1991; Velvizhi et al., 2002). Our results confirm those previously showing that AKG is able to replace dietary dispensable AAs by shunting ammonium back into the dispensable AA pool (Filip and Pierzynowski, 2007; Chen et al., 2017b). Moreover, it has been suggested that N retention is good in infants with a bifidus microflora, Bifidobacterium promotes the amino acids metabolism. The ability of Bifidobacterium to utilize NH3 as a source of N may also lead to decrease of NH3 in the colon (Arunachalam, 1999). Therefore, we speculate that the decreasing of NH3 could be also associated with increasing proportions of Bifidobacterium in the current study.
Conclusion
We investigated the effects on growth performance, cecal microbial communities, and parameters of microbial metabolism in the cecum when pigs were fed a LP diet supplemented with AKG. In particular, this addition altered microbial communities by increasing of the SCFA-producing bacteria and the amounts of Bacteroides and Bifidobacterium, while also decreasing Escherichia coli and the concentration of ammonia in those tissues, thereby increasing ADG of growing pigs. These findings may help us understand the mechanisms by which AKG improves pig performance, and provide additional information that might support the future use of this supplement in animal production.
Author Contributions
JC and KY conceived the experiment and revised the manuscript. JC, QJ, MH, and BK performed the experiments. JC, QJ, CF, LL, and BK provided the reagents and experimental materials. JC analyzed the data and wrote the manuscript. LL, YZ, and CF prepared the figures and edited the manuscript. All authors reviewed the manuscript.
Funding
This work was supported by the National Natural Science Foundation of China (31472107); the Youth Science Fund Project of the National Natural Science Foundation of China (31702126); the Chinese Academy of Sciences ‘Hundred Talent’ award, the National Science Foundation for Distinguished Young Scholars of Hunan Province (2016JJ1015); the Postgraduate Research and Innovation Project of Hunan Province (CX2017B348); the Hunan Province “Hunan Young Science and Technology Innovation Talent” Project (2015RS4053); the Hunan Agricultural University Provincial Outstanding Doctoral Dissertation Cultivating Fund (YB2017002), and the Open Foundation of Key Laboratory of Agro-ecological Processes in Subtropical Region, Institute of Subtropical Agriculture, Chinese Academy of Sciences (ISA2016101).
Conflict of Interest Statement
The authors declare that the research was conducted in the absence of any commercial or financial relationships that could be construed as a potential conflict of interest.
Acknowledgments
We would like to thank the workers in Novogene Bioinformatics Technology Co. (Beijing, China), and thank Dr. Jia Yin and Huansheng Yang at Animal Nutrition and Human Health Laboratory, School of Life Sciences, Hunan Normal University (Changsha, Hunan 410081, China) for their technical assistance. We are also grateful for the support of Livestock Ecology and Healthy Production Team of Institute of Subtropical Agriculture, Chinese Academy of Sciences.
Supplementary Material
The Supplementary Material for this article can be found online at: https://www.frontiersin.org/articles/10.3389/fmicb.2018.01057/full#supplementary-material
Footnotes
- ^ http://ccb.jhu.edu/software/FLASH/
- ^ http://qiime.org/index.html
- ^ http://www.drive5.com/usearch/manual/uchime_algo.html
- ^ http://drive5.com/uparse/
- ^ http://sourceforge.net/projects/rdp-classifier/
- ^ http://greengenes.lbl.gov
References
Arunachalam, K. D. (1999). Role of Bifidobacteria in nutrition, medicine and technology. Nutr. Res. 19, 1559–1597. doi: 10.1016/S0271-5317(99)00112-8
Canh, T. T., Aarnink, A. J. A., Schutte, J. B., Sutton, A., Langhout, D. J., and Verstegen, M. W. A. (1998). Dietary protein affects nitrogen excretion and ammonia emission from slurry of growing-finishing pigs. Livest. Prod. Sci. 56, 181–191. doi: 10.1016/S0301-6226(98)00156-0
Cani, P. D., and Delzenne, N. M. (2009). The role of the gut microbiota in energy metabolism and metabolic disease. Curr. Pharm. Des. 15, 1546–1558. doi: 10.2174/138161209788168164
Caporaso, J. G., Kuczynski, J., Stombaugh, J., Bittinger, K., Bushman, F. D., Costello, E. K., et al. (2010). QIIME allows analysis of high-throughput community sequencing data. Nat. Methods 7, 335–336. doi: 10.1038/nmeth.f.303
Caporaso, J. G., Lauber, C. L., Walters, W. A., Berglyons, D., Lozupone, C. A., Turnbaugh, P. J., et al. (2011). Global patterns of 16S rRNA diversity at a depth of millions of sequences per sample. Proc. Natl. Acad. Sci. U.S.A. 108, 4516–4522. doi: 10.1073/pnas.1000080107
Chen, J. S., Wu, F., Yang, H. S., Li, F. N., Jiang, Q., Liu, S. J., et al. (2017a). Growth performance, nitrogen balance, and metabolism of calcium and phosphorus in growing pigs fed diets supplemented with alpha-ketoglutarate. Anim. Feed Sci. Technol. 226, 21–28. doi: 10.1016/j.anifeedsci.2016.12.013
Chen, J. S., Yang, H. S., Long, L. N., Zhao, Y. R., Jiang, Q., and Wu, F. (2017b). The effects of dietary supplementation with α-ketoglutarate on the intestinal microbiota, metabolic profiles, and ammonia levels in growing pigs. Anim. Feed Sci. Technol. 234, 321–328. doi: 10.1016/j.anifeedsci.2017.03.017
Dai, Z. L., Jing, Z., Wu, G., and Zhu, W. Y. (2010). Utilization of amino acids by bacteria from the pig small intestine. Amino Acids 39, 1201–1215. doi: 10.1007/s00726-010-0556-9
Dai, Z. L., Li, X. L., Xi, P. B., Zhang, J., Wu, G., and Zhu, W. Y. (2012). Regulatory role for l -arginine in the utilization of amino acids by pig small-intestinal bacteria. Amino Acids 43, 233–244. doi: 10.1007/s00726-011-1067-z
DeSantis, T. Z., Hugenholtz, P., Larsen, N., Rojas, M., Brodie, E. L., Keller, K., et al. (2006). Greengenes, a chimera-checked 16S rRNA gene database and workbench compatible with ARB. Appl. Environ. Microbiol. 72, 5069–5072. doi: 10.1128/AEM.03006-05
Diao, H., Zheng, P., Yu, B., He, J., Mao, X. B., Yu, J., et al. (2014). Effects of dietary supplementation with benzoic acid on intestinal morphological structure and microflora in weaned piglets. Livest. Sci. 167, 249–256. doi: 10.1016/j.livsci.2014.05.029
Dong, X., Wei, Y., Yu, J., Wang, Y., and Xu, Q. (2014). Glutamine precursor supplementation increases glutamine synthetase gene expression in intestine of common carp (Cyprinus carpio). Aquac. Res. 45, 1559–1566. doi: 10.1111/are.12354
Dyck, J. R., Kudo, N., Barr, A. J., Davies, S. P., Hardie, D. G., and Lopaschuk, G. D. (1999). Phosphorylation control of cardiac acetyl-CoA carboxylase by cAMP-dependent protein kinase and 5′-AMP activated protein kinase. Eur. J. Biochem. 262, 184–190. doi: 10.1046/j.1432-1327.1999.00371.x
Edgar, R. C. (2013). UPARSE: highly accurate OTU sequences from microbial amplicon reads. Nat. Methods 10, 996–998. doi: 10.1038/nmeth.2604
Edgar, R. C., Haas, B. J., Clemente, J. C., Quince, C., and Knight, R. (2011). UCHIME improves sensitivity and speed of chimera detection. Bioinformatics 27, 2194–2200. doi: 10.1093/bioinformatics/btr381
Filip, R., and Pierzynowski, S. G. (2007). The role of glutamine and a-ketoglutarate in gut metabolism and the potential application in medicine and nutrition. J. Clin. Res. 1, 9–15.
Fleury, M. A., Jouy, E., Eono, F., Cariolet, R., Couet, W., Gobin, P., et al. (2016). Impact of two different colistin dosing strategies on healthy piglet fecal microbiota. Res. Vet. Sci. 107, 152–160. doi: 10.1016/j.rvsc.2016.06.003
Geypens, B., Claus, D., Evenepoel, P., Hiele, M., Maes, B., Peeters, M., et al. (1997). Influence of dietary protein supplements on the formation of bacterial metabolites in the colon. Gut 41, 70–76. doi: 10.1136/gut.41.1.70
Giannenas, I., Doukas, D., Karamoutsios, A., Tzora, A., Bonos, E., Skoufos, I., et al. (2016). Effects of enterococcus faecium, mannan oligosaccharide, benzoic acid and their mixture on growth performance, intestinal microbiota, intestinal morphology and blood lymphocyte subpopulations of fattening pigs. Anim. Feed Sci. Technol. 220, 159–167. doi: 10.1016/j.anifeedsci.2016.08.003
Halas, D., Hansen, C. F., Hampson, D. J., Mullan, B. P., Kim, J. C., Wilson, R. H., et al. (2010). Dietary supplementation with benzoic acid improves apparent ileal digestibility of total nitrogen and increases villous height and caecal microbial diversity in weaner pigs. Anim. Feed Sci. Technol. 160, 137–147. doi: 10.1016/j.anifeedsci.2010.07.001
Hansen, C. F., Sørensen, G., and Lyngbye, M. (2007). Reduced diet crude protein level, benzoic acid and inulin reduced ammonia, but failed to influence odour emission from finishing pigs. Livest. Sci. 109, 228–231. doi: 10.1016/j.livsci.2007.01.133
Hayes, E. T., Leek, A. B., Curran, T. P., Dodd, V. A., Carton, O. T., Beattie, V. E., et al. (2004). The influence of diet crude protein level on odour and ammonia emissions from finishing pig houses. Bioresour. Technol. 91, 309–315. doi: 10.1016/S0960-8524(03)00184-6
He, Q., Kong, X., Wu, G., Ren, P., Tang, H., Hao, F., et al. (2009). Metabolomic analysis of the response of growing pigs to dietary L-arginine supplementation. Amino Acids 37, 199–208. doi: 10.1007/s00726-008-0192-9
Hill, J. E., Hemmingsen, S. M., Goldade, B. G., Dumonceaux, T. J., Klassen, J., Zijlstra, R. T., et al. (2005). Comparison of ileum microflora of pigs fed corn-, wheat-, or barley-based diets by chaperonin-60 sequencing and quantitative PCR. Appl. Environ. Microbiol. 71, 867–875. doi: 10.1128/AEM.71.2.867-875.2005
Hou, Y., Lei, W., Ding, B., Liu, Y., Zhu, H., Jian, L., et al. (2010). Dietary α-ketoglutarate supplementation ameliorates intestinal injury in lipopolysaccharide-challenged piglets. Amino Acids 39, 555–564. doi: 10.1007/s00726-010-0473-y
Hou, Y., Wang, L., Ding, B., Liu, Y., Zhu, H., Liu, J., et al. (2011a). Alpha-ketoglutarate and intestinal function. Front. Biosci. 16, 1186–1196. doi: 10.2741/3783
Hou, Y., Yao, K., Wang, L., Ding, B., Fu, D., Liu, Y., et al. (2011b). Effects of α-ketoglutarate on energy status in the intestinal mucosa of weaned piglets chronically challenged with lipopolysaccharide. Br. J. Nutr. 106, 357–363. doi: 10.1017/S0007114511000249
Isaacson, R., and Kim, H. B. (2012). The intestinal microbiome of the pig. Anim. Health Res. Rev. 13, 100–109. doi: 10.1017/S1466252312000084
Ji, Y., Kong, X., Li, H., Qian, Z., Guo, Q., and Yin, Y. (2017). Effects of dietary nutrient levels on microbial community composition and diversity in the ileal contents of pregnant Huanjiang mini-pigs. PLoS One 12:e0172086. doi: 10.1371/journal.pone.0172086
Junghans, P., Derno, M., Pierzynowski, S., Hennig, U., Eberhard, P., and Souffrant, W. (2006). Intraduodenal infusion of α -ketoglutarate decreases whole body energy expenditure in growing pigs [J]. Clin. Nutr. 25, 489–496. doi: 10.1016/j.clnu.2005.11.003
Kazempour, F., and Jahanian, R. (2017). Effects of different organic acids on performance, ileal microflora, and phosphorus utilization in laying hens fed a diet deficient in non-phytate phosphorus. Anim. Feed Sci. Technol. 223, 110–118. doi: 10.1016/j.anifeedsci.2016.11.006
Kim, H. B., Borewicz, K., White, B. A., Singer, R. S., Sreevatsan, S., Tu, Z. J., et al. (2011). Longitudinal investigation of the age-related bacterial diversity in the feces of commercial pigs. Vet. Microbiol. 153, 124–133. doi: 10.1016/j.vetmic.2011.05.021
Kluge, H., Broz, J., and Eder, K. (2006). Effect of benzoic acid on growth performance, nutrient digestibility, nitrogen balance, gastrointestinal microflora and parameters of microbial metabolism in piglets. J. Anim. Physiol. Anim. Nutr. 90, 316–324. doi: 10.1111/j.1439-0396.2005.00604.x
Knarreborg, A., Miquel, N., Granli, T., and Jensen, B. B. (2002). Establishment and application of an in vitro methodology to study the effects of organic acids on coliform and lactic acid bacteria in the proximal part of the gastrointestinal tract of piglets. Anim. Feed Sci. Technol. 99, 131–140. doi: 10.1016/S0377-8401(02)00069-X
Kobayashi, H., Nakashima, K., Ishida, A., Ashihara, A., and Katsumata, M. (2013). Effects of low protein diet and low protein diet supplemented with synthetic essential amino acids on meat quality of broiler chickens. Anim. Sci. J. 84, 489–495. doi: 10.1111/asj.12021
Kong, X. F., Zhang, Y. Z., Wu, X., Yin, Y. L., Tan, Z. L., Feng, Y., et al. (2009). Fermentation characterization of Chinese yam polysaccharide and its effects on the gut microbiota of rats. Int. J. Microbiol. 2009, 1–13. doi: 10.1155/2009/598152
Kong, X. F., Zhou, X. L., Lian, G. Q., Blachier, F., Liu, G., Tan, B. E., et al. (2014). Dietary supplementation with chitooligosaccharides alters gut microbiota and modifies intestinal luminal metabolites in weaned Huanjiang mini-piglets. Livest. Sci. 160, 97–101. doi: 10.1016/j.livsci.2013.11.023
Konstantinov, S. R., Awati, A. A., Williams, B. A., Miller, B. G., Jones, P., Stokes, C. R., et al. (2006). Post-natal development of the porcine microbiota composition and activities. Environ. Microbiol. 8, 1191–1199. doi: 10.1111/j.1462-2920.2006.01009.x
Kraler, M., Ghanbari, M., Domig, K. J., Schedle, K., and Kneifel, W. (2016). The intestinal microbiota of piglets fed with wheat bran variants as characterised by 16S rRNA next-generation amplicon sequencing. Arch. Anim. Nutr. 70, 173–189. doi: 10.1080/1745039X.2016.1160534
Lambert, B. D., Filip, R., Stoll, B., Junghans, P., Derno, M., Hennig, U., et al. (2006). First-pass metabolism limits the intestinal absorption of enteral alpha-ketoglutarate in young pigs. J. Nutr. 136, 2779–2784. doi: 10.1093/jn/136.11.2779
Lambert, B. D., Stoll, B., Niinikoski, H., Pierzynowski, S., and Burrin, D. G. (2002). Net portal absorption of enterally fed alpha-ketoglutarate is limited in young pigs. J. Nutr. 132, 3383–3386. doi: 10.1093/jn/132.11.3383
Le Bellego, L., and Noblet, J. (2002). Performance and utilization of dietary energy and amino acids in piglets fed low protein diets. Livest. Prod. Sci. 76, 45–58. doi: 10.1016/S0301-6226(02)00008-8
Liem, A., Pesti, G. M., and Edwards, H. M. Jr. (2008). The effect of several organic acids on phytate phosphorus hydrolysis in broiler chicks. Poult. Sci. 87, 689–693. doi: 10.3382/ps.2007-00256
Luo, Z., Li, C., Cheng, Y., Hang, S., and Zhu, W. (2015). Effects of low dietary protein on the metabolites and microbial communities in the caecal digesta of piglets. Arch. Anim. Nutr. 69, 212–226. doi: 10.1080/1745039X.2015.1034521
Magoč, T., and Salzberg, S. L. (2011). FLASH: fast length adjustment of short reads to improve genome assemblies. Bioinformatics 27, 2957–2963. doi: 10.1093/bioinformatics/btr507
Metges, C. C. (2000). Contribution of microbial amino acids to amino acid homeostasis of the host. J. Nutr. 130, 1857S–1864S. doi: 10.1093/jn/130.7.1857S
Metzlerzebeli, B. U., Schmitzesser, S., Mann, E., Grüll, D., Molnar, T., and Zebeli, Q. (2015). Adaptation of the cecal bacterial microbiome of growing pigs in response to resistant starch type 4. Appl. Environ. Microbiol. 81, 8489–8499. doi: 10.1128/AEM.02756-15
Murphy, P., Dal Bello, F., O’Doherty, J., Arendt, E. K., Sweeney, T., and Coffey, A. (2013). Analysis of bacterial community shifts in the gastrointestinal tract of pigs fed diets supplemented with beta-glucan from Laminaria digitata, Laminaria hyperborea, and Saccharomyces cerevisiae. Animal 7, 1079–1087. doi: 10.1017/S1751731113000165
Muyzer, G., de Waal, E. C., and Uitterlinden, A. G. (1993). Profiling of complex microbial populations by denaturing gradient gel electrophoresis analysis of polymerase chain reaction-amplified genes coding for 16S rRNA. Appl. Environ. Microbiol. 59, 695–700.
Nyangale, E. P., Mottram, D. S., and Gibson, G. R. (2015). Gut microbial activity, implications for health and disease: the potential role of metabolite analysis. J. Proteome Res. 11, 5573–5585. doi: 10.1021/pr300637d
Olukosi, O. A., and Dono, N. D. (2014). Modification of digesta pH and intestinal morphology with the use of benzoic acid or phytobiotics and the effects onbroiler chicken growth performance and energy and nutrient utilization. J. Anim. Sci. 92, 3945–3953. doi: 10.2527/jas.2013-6368
Opapeju, F. O., Rademacher, M., Blank, G., and Nyachoti, C. M. (2008). Effect of low-protein amino acid-supplemented diets on the growth performance, gut morphology, organ weights and digesta characteristics of weaned pigs. Animal 2, 1457–1464. doi: 10.1017/S175173110800270X
Øverland, M., Kjos, N. P., Borg, M., Skjerve, E., and Sørum, H. (2008). Organic acids in diets for entire male pigs: effect on skatole level, microbiota in digesta, and growth performance. Livest. Sci. 115, 169–178. doi: 10.1016/j.livsci.2007.07.007
Partanen, K. H., and Mroz, Z. (1999). Organic acids for performance enhancement in pig diets. Nutr. Res. Rev. 12, 117–145. doi: 10.1079/095442299108728884
Penders, J., Thijs, C., Vink, C., Stelma, F. F., Snijders, B., Kummeling, I., et al. (2006). Factors influencing the composition of the intestinal microbiota in early infancy. Pediatrics 118, 511–521. doi: 10.1542/peds.2005-2824
Pieper, R., Vahjen, W., Neumann, K., Van Kessel, A. G., and Zentek, J. (2012). Dose-dependent effects of dietary zinc oxide on bacterial communities and metabolic profiles in the ileum of weaned pigs. J. Anim. Physiol. Anim. Nutr. 96, 825–833. doi: 10.1111/j.1439-0396.2011.01231.x
Piva, A., Casadei, G., and Biagi, G. (2002). An organic acid blend can modulate swine intestinal fermentation and reduce microbial proteolysis. Can. J. Anim. Sci. 82, 527–532. doi: 10.4141/A01-090
Prandini, A., Morlacchini, M., Sigolo, S., and Fiorentini, L. (2012). Anticatabolic activity of alpha-ketoglutaric acid in growing rats. Ital. J. Anim. Sci. 11, 279–284. doi: 10.4081/ijas.2012.e52
Qi, H., Xiang, Z., Han, G., Yu, B., Huang, Z., and Chen, D. (2011). Effects of different dietary protein sources on cecal microflora in rats. Afr. J. Biotechnol. 10, 3704–3708. doi: 10.5897/AJB10.2677
Singh, P., Teal, T. K., Marsh, T. L., Tiedje, J. M., Mosci, R., Jernigan, K., et al. (2015). Intestinal microbial communities associated with acute enteric infections and disease recovery. Microbiome 3:45. doi: 10.1186/s40168-015-0109-2
Stoll, B., McNelly, S., Buscher, H. P., and Häussinger, D. (1991). Functional hepatocyte heterogeneity in glutamate, aspartate and alpha-ketoglutarate uptake: a histoautoradiographical study. Hepatology 13, 247–253. doi: 10.1002/hep.1840130208
Su, Y., Yao, W., Perez-Gutierrez, O. N., Smidt, H., and Zhu, W. Y. (2008). 16S ribosomal RNA-based methods to monitor changes in the hindgut bacterial community of piglets after oral administration of Lactobacillus sobrius S1. Anaerobe 14, 78–86. doi: 10.1016/j.anaerobe.2007.12.004
Tennyson, C. A., and Friedman, G. (2008). Microecology, obesity, and probiotics. Curr. Opin. Endocrinol. Diabetes Obes. 15, 422–427. doi: 10.1097/MED.0b013e328308dbfb
Velvizhi, S., Dakshayani, K. B., and Subramanian, P. (2002). Effects of α-ketoglutarate on antioxidants and lipid peroxidation products in rats treated with ammonium acetate. Nutrition 18, 747–750. doi: 10.1016/S0899-9007(02)00825-0
Walsh, M. C., Sholly, D. M., Hinson, R. B., Saddoris, K. L., Sutton, A. L., Radcliffe, J. S., et al. (2007). Effects of water and diet acidification with and without antibiotics on weanling pig growth and microbial shedding. J. Anim. Sci. 85, 1799–1808. doi: 10.2527/jas.2006-049
Wang, L., Wei, Y., Wang, C., Li, J., Zhao, Z., Luo, L., et al. (2016). Effects of α-ketoglutarate on the growth performance, amino acid metabolism and related gene expression of mirror carp (Cyprinus carpio). Aquac. Nutr. 23, 926–933. doi: 10.1111/anu.12460
Wang, L. S., Xu, Q. Y., Wang, C. A., Li, J. N., Chen, D., Zhao, Z. G., et al. (2015). Effects of dietary α-ketoglutarate supplementation on the growth performance, glutamine synthesis and amino acid concentrations of juvenile hybrid sturgeon Acipenser schrenckii × A. baerii fed high levels of soy protein concentrate. Anim. Feed Sci. Technol. 211, 199–207. doi: 10.1016/j.anifeedsci.2015.11.016
Wang, Q., Garrity, G. M., Tiedje, J. M., and Cole, J. R. (2007). Naive Bayesian classifier for rapid assignment of rRNA sequences into the new bacterial taxonomy. Appl. Environ. Microbiol. 73, 5261–5267. doi: 10.1128/AEM.00062-07
Williams, B. A., Zhang, D., Lisle, A. T., Mikkelsen, D., McSweeney, C. S., Kang, S., et al. (2015). Soluble arabinoxylan enhances large intestinal microbial health biomarkers in pigs fed a red meat-containing diet. Nutrition 32, 491–497. doi: 10.1016/j.nut.2015.10.008
Wirén, M., Permert, J., and Larsson, J. (2002). Alpha-ketoglutarate-supplemented enteral nutrition: effects on postoperative nitrogen balance and muscle catabolism. Nutrition 18, 725–728. doi: 10.1016/S0899-9007(02)00844-4
Yan, H., Potu, R., Lu, H., Vezzoni de Almeida, V., Stewart, T., Ragland, D., et al. (2012). Dietary fat content and fiber type modulate hind gut microbial community and metabolic markers in the pig. PLoS One 8:e59581. doi: 10.1371/journal.pone.0059581
Yao, K., Yin, Y. L., Li, X. L., Xi, P. B., Wang, J. J., Lei, J., et al. (2012). Alpha-ketoglutarate inhibits glutamine degradation and enhances protein synthesis in intestinal porcine epithelial cells. Amino Acids 42, 2491–2500. doi: 10.1007/s00726-011-1060-6
Zhang, C., Yu, M., Yang, Y., Mu, C., Su, Y., and Zhu, W. (2016). Effect of early antibiotic administration on cecal bacterial communities and their metabolic profiles in pigs fed diets with different protein levels. Anaerobe 42, 188–196. doi: 10.1016/j.anaerobe.2016.10.016
Zhang, H. L., Ji, S. K., Chen, Y., Yang, B., Xu, X. L., and Hu, C. J. (2011). Effect of alpha-ketoglutaric acid on in vitro gas production, ruminal fermentation, and bacterial diversity. Anim. Feed Sci. Technol. 170, 291–296. doi: 10.1016/j.anifeedsci.2011.09.012
Zhou, D., Pan, Q., Shen, F., Cao, H. X., Ding, W. J., Chen, Y. W., et al. (2017). Total fecal microbiota transplantation alleviates high-fat diet-induced steatohepatitis in mice via beneficial regulation of gut microbiota. Sci. Rep. 7:1529. doi: 10.1038/s41598-017-01751-y
Keywords: alpha-ketoglutarate, low-protein diet, cecal, bacterial communities, metabolites, growing pigs
Citation: Chen J, Kang B, Jiang Q, Han M, Zhao Y, Long L, Fu C and Yao K (2018) Alpha-Ketoglutarate in Low-Protein Diets for Growing Pigs: Effects on Cecal Microbial Communities and Parameters of Microbial Metabolism. Front. Microbiol. 9:1057. doi: 10.3389/fmicb.2018.01057
Received: 09 July 2017; Accepted: 04 May 2018;
Published: 31 May 2018.
Edited by:
M. Pilar Francino, Fundación para el Fomento de la Investigación Sanitaria y Biomédica de la Comunitat Valenciana (FISABIO), SpainReviewed by:
Alfonso Benítez-Páez, Instituto de Agroquímica y Tecnología de Alimentos (IATA), SpainLuis David Alcaraz, Universidad Nacional Autónoma de México, Mexico
Copyright © 2018 Chen, Kang, Jiang, Han, Zhao, Long, Fu and Yao. This is an open-access article distributed under the terms of the Creative Commons Attribution License (CC BY). The use, distribution or reproduction in other forums is permitted, provided the original author(s) and the copyright owner are credited and that the original publication in this journal is cited, in accordance with accepted academic practice. No use, distribution or reproduction is permitted which does not comply with these terms.
*Correspondence: Chenxing Fu, chenxing1110@gmail.com Kang Yao, yaokang@isa.ac.cn