Dopamine-Induced Changes in Gαolf Protein Levels in Striatonigral and Striatopallidal Medium Spiny Neurons Underlie the Genesis of L-DOPA-Induced Dyskinesia in Parkinsonian Mice
- 1Department of Neurodegenerative Disorders Research, Institute of Biomedical Sciences, Graduate School of Medical Sciences, Tokushima University, Tokushima, Japan
- 2Parkinson’s Disease and Dystonia Research Center, Tokushima University Hospital, Tokushima, Japan
- 3Department of Neurosurgery, Institute of Biomedical Sciences, Graduate School of Medical Sciences, Tokushima University, Tokushima, Japan
The dopamine precursor, L-3,4-dihydroxyphenylalanine (L-DOPA), exerts powerful therapeutic effects but eventually generates L-DOPA-induced dyskinesia (LID) in patients with Parkinson’s disease (PD). LID has a close link with deregulation of striatal dopamine/cAMP signaling, which is integrated by medium spiny neurons (MSNs). Olfactory type G-protein α subunit (Gαolf), a stimulatory GTP-binding protein encoded by the GNAL gene, is highly concentrated in the striatum, where it positively couples with dopamine D1 (D1R) receptor and adenosine A2A receptor (A2AR) to increase intracellular cAMP levels in MSNs. In the striatum, D1Rs are mainly expressed in the MSNs that form the striatonigral pathway, while D2Rs and A2ARs are expressed in the MSNs that form the striatopallidal pathway. Here, we examined the association between striatal Gαolf protein levels and the development of LID. We used a hemi-parkinsonian mouse model with nigrostriatal lesions induced by 6-hydroxydopamine (6-OHDA). Using quantitative immunohistochemistry (IHC) and a dual-antigen recognition in situ proximity ligation assay (PLA), we here found that in the dopamine-depleted striatum, there appeared increased and decreased levels of Gαolf protein in striatonigral and striatopallidal MSNs, respectively, after a daily pulsatile administration of L-DOPA. This leads to increased responsiveness to dopamine stimulation in both striatonigral and striatopallidal MSNs. Because Gαolf protein levels serve as a determinant of cAMP signal-dependent activity in striatal MSNs, we suggest that L-DOPA-induced changes in striatal Gαolf levels in the dopamine-depleted striatum could be a key event in generating LID.
Introduction
Human pathology has shown that Parkinson’s disease (PD) results from dopamine deficiency in the neostriatum, particularly in the putamen, due to degenerative loss of nigrostriatal dopaminergic cells (Kish et al., 1988; Goto et al., 1989). Treatments with the dopamine precursor, L-3,4-dihydroxyphenylalanine (L-DOPA), remain the gold standard of drug therapy for PD. However, after prolonged and pulsatile exposure to L-DOPA, PD patients eventually develop L-DOPA-induced dyskinesia (LID; Jenner, 2008; Calabresi et al., 2010; Huot et al., 2013). LID is an adverse event that occurs in more than 50% of patients after 5–10 years (Ahlskog and Muenter, 2001; Rascol et al., 2006). Importantly, once LID has been established, its severity increases unless dopaminergic drug dosage is reduced (Brotchie, 2005). It is known that the severity of loss of nigral dopaminergic cells represents the most important factor that determines the severity of LID (Guridi et al., 2012; Bastide et al., 2015). However, the nature of the cellular and molecular key events that lead to a progressive increase in responsiveness to dopaminergic stimulation in LID remains unclear.
LID is closely linked with pathological changes in dopaminergic transmissions in the striatum (Bastide et al., 2015; Calabresi et al., 2016). Dopamine receptors are categorized into two subclasses, D1- and D2-type receptors, based on their functional properties to stimulate and inhibit the adenylyl cyclase-mediated cAMP production via specific targeting of G-proteins, respectively (Kebabian and Calne, 1979; Missale et al., 1998). There is a large body of evidence showing that increased activity of dopamine D1-receptors (D1Rs) is necessary for LID development (Westin et al., 2007; Darmopil et al., 2009; Alcacer et al., 2012). D1R activation leads to multiple molecular events, such as the induction of immediate early genes (Cenci et al., 1999; Gerfen et al., 2002; Darmopil et al., 2009) and the activation of extracellular signal-regulated kinases (Gerfen et al., 2002; Pavón et al., 2006; Santini et al., 2007, 2009; Westin et al., 2007; Rylander et al., 2009; Ding et al., 2011). Striatal dopamine/cAMP signaling is integrated by medium spiny neurons (MSNs), which are the principal neurons of the striatum (Graybiel, 2008; Kreitzer, 2009; Gerfen and Surmeier, 2011). MSNs can be divided into two distinct subpopulations on the basis of their axon projections, which form the “direct” striatonigral and “indirect” striatopallidal pathways (Crittenden and Graybiel, 2011; Gerfen and Surmeier, 2011). Interestingly, anatomical evidence has shown that D1Rs and D2Rs are mainly expressed in striatonigral and striatopallidal MSNs, respectively. Moreover, adenosine A2A receptor (A2AR), a prototypical Gs-coupled receptor, is enriched in the striatum, where it is mainly expressed in striatopallidal, but not striatonigral, MSNs (Schiffmann et al., 1991; Svenningsson et al., 1999; Schwarzschild et al., 2006; Fuxe et al., 2007).
Olfactory type G-protein α subunit (Gαolf), the stimulatory G-protein encoded by the GNAL gene, is highly concentrated in the striatum, where it positively couples with D1R and A2AR to activate adenylyl cyclase and, thereby, increase intracellular cAMP levels in MSNs (Hervé, 2011). As Gαolf represents the rate-limiting factor for the D1R- and A2AR-dependent cAMP production (Kull et al., 2000; Corvol et al., 2001), Gαolf protein level serves as a determinant of cAMP signal-dependent activity in both D1R-expressing striatonigral MSNs (D1-cells) and D2R-expressing striatopallidal MSNs (D2-cells). D1R/Gαolf-mediated increases in intracellular cAMP levels facilitate D1-cell activity (Hervé, 2011), while the elevation of intracellular cAMP levels via A2AR/Gαolf activation functionally opposes the actions of D2Rs on D2-cells (Schwarzschild et al., 2006; Fuxe et al., 2007). It is also known that Gαolf protein levels in striatal MSNs are regulated by posttranslational usage-dependent mechanism through the activation of D1Rs (Hervé et al., 2001; Corvol et al., 2004, 2007; Alcacer et al., 2012; Ruiz-DeDiego et al., 2015) and A2ARs (Hervé et al., 2001).
The aim of this study was to clarify the association of striatal Gαolf protein levels with LID development. For this purpose, we used a hemi-parkinsonian mouse model with nigrostriatal lesion induced by 6-hydroxydopamine (6-OHDA). Using quantitative immunohistochemistry (IHC) and a highly-sensitive in situ proximity ligation assay (PLA), we show that in the 6-OHDA-lesioned striatum, daily pulsatile injections of L-DOPA might cause changes in Gαolf levels in not only D1-cells but also D2-cells, and lead to elevated responsiveness to dopamine stimulation in both D1-cells and D2-cells. This novel finding suggests that L-DOPA-induced changes in striatal Gαolf levels in the dopamine-denervated striatum may serve as a principal cause for generating LID.
Materials and Methods
Experimental Animals
All experimental procedures involving the use of animals and the analysis of brain anatomy were approved by the Institutional Care and Use Committees of Tokushima University, Japan. Adult male C57BL/6 mice aged 8–9 weeks were purchased from Nihon SLC Co. (Shizuoka, Japan). Mice were housed in a controlled environment (23 ± 1°C, 50 ± 5% of humidity) with 12 h light/dark cycle. Mice were allowed to take food and tap water ad libitum.
Stereotaxic Injection of 6-OHDA
Mice were anesthetized with isoflurane (Sigma-Aldrich, St. Louis, MO, USA) and were mounted on a stereotaxic frame (Narishige, Tokyo, Japan). Each mouse received a stereotaxic injection of 6-OHDA-HCl (8.2 μg) dissolved in 4 μl of saline containing 0.02% ascorbic acid. Two 2-μl injections were administered into the striatum at a rate of 1 μl/min. The needle was left in place for 5 min to allow diffusion away from the injection site. The stereotaxic coordinates according to the mouse brain atlas (Paxinos and Franklin, 2001) were anterior-posterior, +0.5; medial-lateral, +2.4; and dorsal-ventral, −4.0 and −3.0. Mice were allowed to recover for 3 weeks and then apomorphine (Sigma-Aldrich; 0.5 mg/kg)-induced rotation behavior was studied over the course of 60 min. Mice with contralateral rotations (>7 times/min) were chosen and used for further studies.
L-DOPA Treatments
Three weeks after the 6-OHDA-lesioning, mice received intraperitoneal injections of L-DOPA (Sigma-Aldrich; 20 mg/kg of free base) dissolved in 0.9% saline and intraperitoneal injections of benserazide-HCl (Sigma-Aldrich; 12 mg/kg) dissolved in 0.9% saline 20 min before daily administration of L-DOPA over 10 days. On day 11, the mice underwent behavioral studies and were then sacrificed for histological studies.
Assessment of Abnormal Involuntary Movements (AIMs)
AIM scoring was performed according to previous reports (Cenci et al., 1998; Pavón et al., 2006; Santini et al., 2007). AIM scores were obtained after the last injection of L-DOPA for 1 min every 10 min over a period of 140 min. For the evaluation, each mouse was placed in a glass cylinder (diameter of 12 cm). Purposeless movements were classified on the basis of their topographic distribution. The following four subtypes of AIMs were present: locomotive (tight contralateral turns), axial (twisted posturing of the neck and upper body toward the contralateral side), forelimb (jerky movements of the contralateral forelimb, and/or grabbing movement of the contralateral paw), and orolingual (jaw movements and tongue protrusion toward the contralateral side). Each subtype was scored as follows; 0, absent; 1, occasional; 2, frequent; 3, continuous; 4, continuous, not interrupted by sensory stimuli.
Tissue Preparations
Immediately after the last AIM scoring, the mice were intraperitoneally administered a lethal dose of pentobarbital (Sigma-Aldrich). They were then transcardially perfused with 0.01 M phosphate-buffered saline (PBS) at pH 7.2, followed by cold 4% paraformaldehyde in 0.1 M phosphate buffer at pH 7.2. The brains were removed, post-fixed overnight in the same fixative at 4°C, and stored in a 10–30% sucrose gradient in 0.1 M phosphate buffer at 4°C for cryoprotection. Sixteen-micrometre-thick sections were cut on a cryostat and stored in PBS containing 0.05% NaN3 until use.
IHC
Immunostaining was performed on free-floating sections using the tyramide signal amplification (TSA) method, as in our previous report (Okita et al., 2012). After blocking endogenous peroxidase activity, the sections were incubated in PBS containing 3% bovine serum albumin (BSA) for 60 min. They were then incubated with antibodies against one of the following (diluted in PBS-BSA): Gαolf (rabbit polyclonal, 1:5000; Santa Cruz Biotechnology, Santa Cruz, CA, USA), tyrosine hydroxylase (TH, rabbit polyclonal, 1:100,000) (Sato et al., 2008; Morigaki and Goto, 2016), D1R (mouse monoclonal, 1:5000; Novus Biologicals, Littleton, CO, USA), A2AR (mouse monoclonal, 1:5000; Santa Cruz Biotechnology), D2R (rabbit polyclonal, 1:2000; Merck Millipore, Billerica, MA, USA) or c-Fos (rabbit polyclonal, 1:50,000; Oncogene Science, Cambridge, MA, USA) for 18 h. The bound antibodies were detected using the Histofine Simple Stain Kit (Nichirei, Tokyo, Japan) and the TSA-system with Cyanine3 or Fluorescein (Perkin Elmer, Shelton, CT, USA). For double immunofluorescence staining, the sections stained for Gαolf using Cyanine3 were incubated in 0.1 M glycine-HCl (pH 2.2) at room temperature for 30 min. After rinsing in PBS for 1 h, the sections were then incubated overnight at room temperature in PBS containing 3% BSA and a rabbit polyclonal antibody against the μ-opioid receptor (MOR; 1:20,000; Millipore, Billerica, MA, USA), a mouse monoclonal antibody against D1R (1:5000; Novus Biologicals), or a mouse monoclonal antibody against A2AR (1:5000; Santa Cruz Biotechnology). The bound antibodies were detected using the Histofine Simple Stain Kit (Nichirei) and the TSA-system with Fluorescein (Perkin Elmer).
Dual-Antigen Recognition In Situ PLA
Dual-antigen recognition PLA experiments were conducted using the Brightfield Duolink PLA kit reagents (Sigma-Aldrich) according to the manufacturer’s recommendations with some modifications. Briefly, after blocking endogenous peroxidases in PBS containing 0.1% H2O2 for 30 min, the free-floating sections were incubated in PBS containing 3% normal goat serum for 60 min. They were then incubated in PBS containing 3% normal goat serum and a rabbit polyclonal antibody against Gαolf (1:500; Santa Cruz Biotechnology) in combination with a mouse monoclonal antibody against D1R (1:500; Novus Biologicals) or a mouse monoclonal antibody against A2AR (1:500; Santa Cruz Biotechnology) for 18 h at room temperature. After subsequent secondary labeling with rabbit PLA minus and mouse PLA plus probes, we used the Brightfield Duolink Detection reagents for ligation and amplification and label probe binding according to the manufacturer’s instructions. For final signal visualization, we used the TSA-system with Cyanine3 (Perkin Elmer). After mounting on slides, the stained sections were counterstained with hematoxylin and were cover-slipped using 10% glycerol in PBS.
Digital Imaging and Morphometry
Digital microscopy images were captured using an Olympus BX51 microscope (Olympus, Tokyo, Japan) equipped with a DP40 digital camera (Olympus). They were imported into Adobe Photoshop CS4 and processed digitally. We adjusted contrast, brightness, and color balance. Using an image analyzer (MetaMorph, Molecular Device, Tokyo, Japan), we measured the optical densities of immunoreactive products and PLA signals in the striatum, which were represented by gray levels on non-colored digital images (Sato et al., 2008; Goto et al., 2013; Morigaki and Goto, 2015). Using the same protocol described above, we also measured optical densities of Gαolf-immunoreactive products in the striosome and matrix subfields in the striatal sections double-stained for Gαolf and MOR. We also counted the numbers of neuronal nuclei positive for c-Fos in a 0.5 mm × 0.5 mm field in the striatum and globus pallidus, as in our previous report (Tanabe et al., 2014). These morphometric analyses were carried out in a blind manner.
Statistical Analysis
All experimental values are expressed as means ± SEM. For two-group comparisons, we used a paired two-tailed t-test. Multiple comparisons were analyzed using one-way or two-way analysis of variance (ANOVA), followed by Bonferroni’s post hoc tests for pair wise comparisons. Statistical analyses were performed using Stat View 5.0 (SAS Institute, Cary, NC, USA) software. P-values of less than 0.05 were considered statistically significant.
Results
Generation of a Mouse Model with LID
To model the generation of AIMs in PD following repeated L-DOPA treatments, we employed a well-established PD mouse model in which mice first received unilateral injection of 6-OHDA into the striatum (Santini et al., 2007). After 3 weeks of recovery and an apomorphine test, the mice were subjected to L-DOPA treatment for 10 days according to standardized protocols (Figure 1A). In this study, 6-OHDA-lesioned mice administered daily injections of benserazide-HCl (12 mg/kg) alone for 10 days were designated as “PD” models. Six-OHDA-lesioned mice that received daily injections of L-DOPA (20 mg/kg) and benserazide-HCl (12 mg/kg) for 10 days and finally exhibited LIDs with total AIM scores of more than 20 were designated as “PD with Dyskinesia (PD-D)” models. Among 6-OHDA-lesioned mice that received daily injections of L-DOPA (n = 28), 25 mice (~90%) were grouped into the PD-D model. Mice that received no drug treatment, except for anesthetic drugs, were used as “naïve controls”.
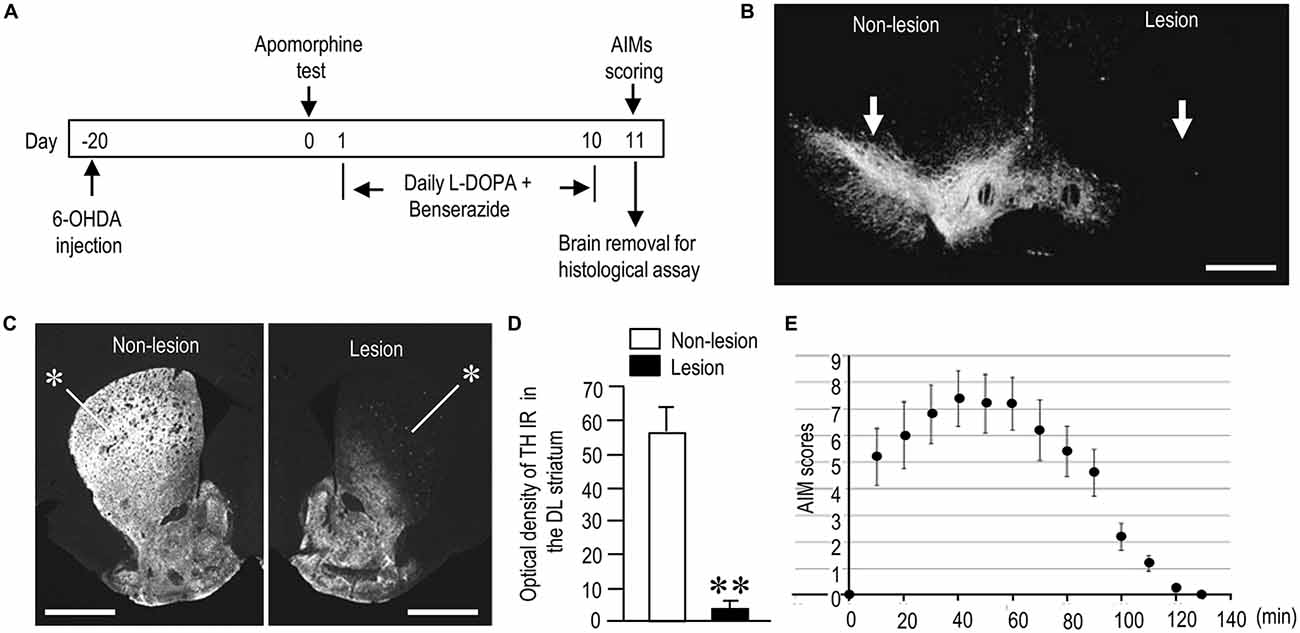
Figure 1. Generation of hemi-parkinsonian mice with L-DOPA-induced dyskinesia (LID). (A) Timeline of treatments and observations (also see “Materials and Methods” Section). (B,C) Representative photomicrographs of the substantia nigra (B, arrows) and striatum (C, asterisks) stained for tyrosine hydroxylase (TH) on the non-lesioned (Non-lesion) and lesioned (Lesion) sides in mice with 6-hydroxydopamine (6-OHDA)-induced lesions. (D) Quantification of mean TH staining intensity in the dorsolateral (DL) striatum on the non-lesioned (Non-lesion) and lesioned (Lesion) sides. Data are means ± SEM (n = 10). Paired two-tailed t test: **P < 0.01 vs. Non-lesion. (E) Time course of total abnormal involuntary movements (AIMs) scored every 10 min over a period of 140 min after the last L-DOPA administration. Data are means ± SEM at the each time point (n = 10 per group). Scale bars: (B) = 1 mm; (C) = 2 mm.
In both PD and PD-D mice, IHC with anti-TH antibody revealed a severe loss of nigral dopaminergic cells (Figure 1B) and striatal dopaminergic afferents (Figure 1C) on the side of the 6-OHDA injection. Quantitative measurements (Figure 1D) revealed a greater-than-90% reduction in TH labeling in the dorsolateral (DL) striatum on the lesioned side when compared to the non-lesioned side (lesion side, 3.9 ± 2.1; non-lesion side, 56.5 ± 8.9; means ± SEM; n = 10; two-tailed t-test, P < 0.01). Figure 1E shows the time course of changes in LIDs as determined by AIM scoring in PD-D mice. AIMs were maximal 40 min after L-DOPA administration, declined after 70 min, and almost disappeared after 120 min.
Regional and Cellular Localization of Gαolf in the Normal Mouse Striatum
Figure 2A depicts the known distributional patterns of Gαolf, D1R, and A2AR in a simplified basal ganglia circuit diagram. Note that Gαolf is mainly localized with D1R in the D1-cells that form the striatonigral pathway, while it is localized with A2AR in the D2-cells that form the striatopallidal pathway. Using IHC, we reappraized the localization profile of Gαolf immunoreactivity (IR) in the mouse striatum. Low-magnification microscopic images show strong Gαolf labeling in the striatum (Figure 2B), particularly in the DL region (Figure 2B’, arrows). As in our previous report (Sako et al., 2010), Gαolf IR was differentially concentrated in the different striatal compartments, with heightened Gαolf labeling in the striosomes relative to the matrix (Figures 2C,D). Optical density measurements (Figure 2E) also revealed that Gαolf IR in the striosomes was significantly higher than that in the matrix (striosomes, 39.8 ± 5.0; matrix, 23.5 ± 6.0; means ± SEM; n = 10; two-tailed t-test, P < 0.01). Microscopic images with high magnification show numerous tiny dots of Gαolf IR densely distributed in the DL striatum (Figures 2F–H). In the double-labeling study, Gαolf-positive dots were frequently localized in MSNs labeled for D1R (Figure 2G) or A2AR (Figure 2H). Using dual-antigen recognition in situ PLA, which indicates that two proteins are in close proximity (Söderberg et al., 2006), we also found that dot signals indicating the presence of Gαolf protein in close proximity to D1R protein (D1R-Gαolf; Figure 2I) or A2AR protein (A2AR-Gαolf; Figure 2J) were abundantly distributed in the DL striatum.
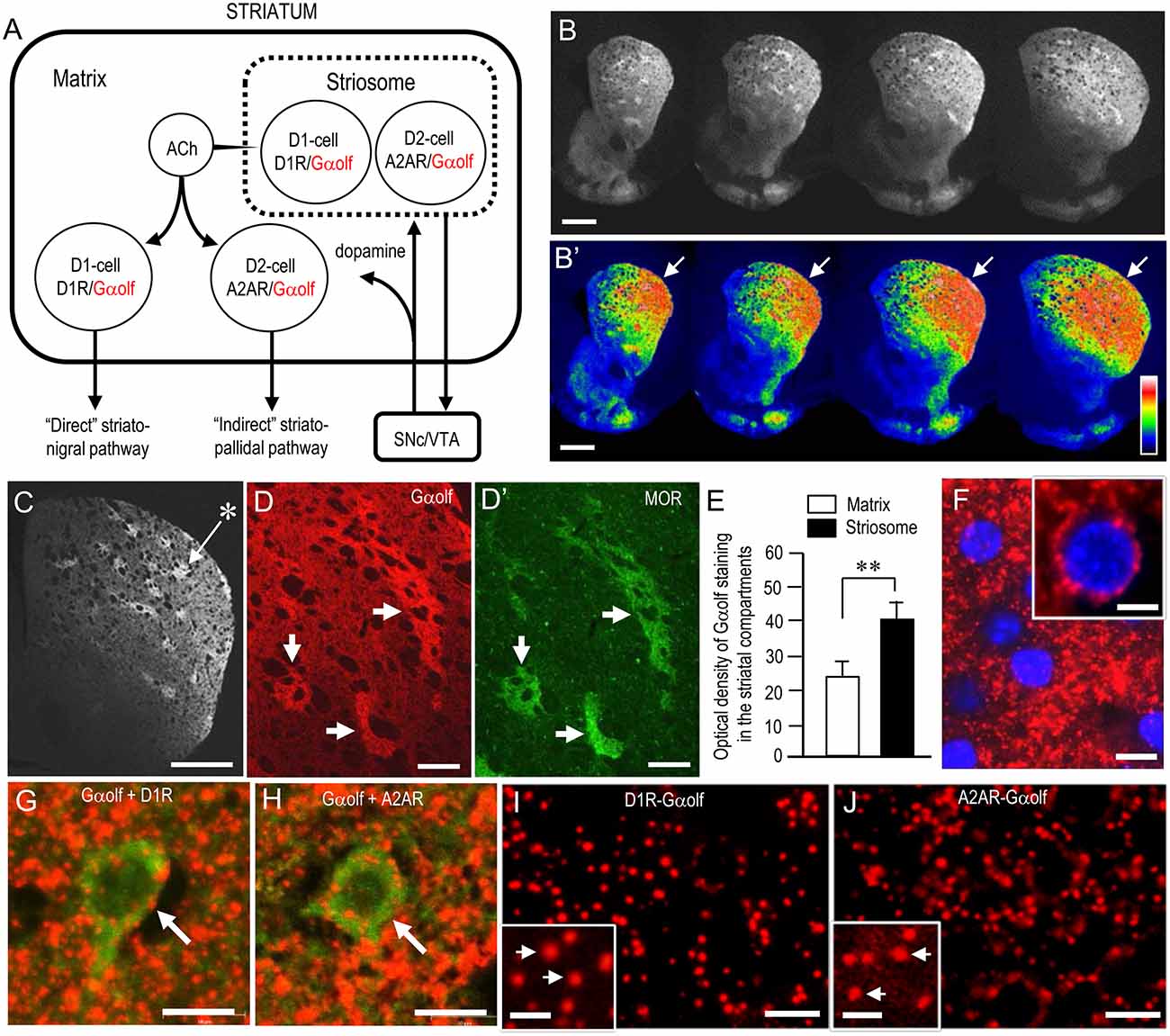
Figure 2. Striatal localization of Gαolf protein in normal mice. (A) Localization pattern of Gαolf in a simplified basal ganglia circuit. Note that Gαolf is colocalized with D1R in striatonigral medium spiny neurons (MSNs; D1-cells), but with adenosine A2A receptor (A2AR) in D2R-expressing striatopallidal MSNs (D2-cells). The striatonigral and striatopallidal pathways arising from the striosome are omitted in this scheme. Abbreviations: SNc, substantia nigra pars compacta; VTA, ventral tegmental area. (B,B’) Multiple frontal sections of the striatum stained for Gαolf from naïve control mice (B) and their graded color-converted images (B’). Note that Gαolf immunoreactivity (IR) is highly concentrated in the DL portion of the striatum (arrows). (C) Representative photomicrograph of the striatum stained for Gαolf. Asterisk indicates an example of the striosomes. (D,D’) Representative photomicrographs of the DL striatum double-stained for Gαolf (D) and μ-opioid receptor (MOR) (D’). Corresponding striosomes are indicated by arrows. (E) Quantification of mean Gαolf staining intensity in the striosome and matrix compartments in the DL striatum. Data are means ± SEM (n = 10). Paired two-tailed Student’s t test: **P < 0.01, Striosome vs. Matrix. (F) Representative photomicrographs of the DL striatum stained for Gαolf with DAPI (4,6-diamidino-2-phenylindole)-staining. Tiny dots positive for Gαolf (inset) are shown. (G,H) Representative photomicrographs of neurons double-stained for Gαolf and D1R (G) or A2AR (H) in the DL striatum. (I,J) Representative photomicrographs of the DL striatum stained with the dual recognition in situ proximity ligation assay (PLA) for Gαolf-D1R (I) or Gαolf-A2AR (J). Tiny dots showing the PLA signals for Gαolf-D1R (I) or Gαolf-A2AR (J) are abundant. Microscopic images at higher magnifications are shown in the insets (arrows) in (I,J). Scale bars: (B,C) = 1 mm; (D,D’) = 200 μm: (F–J) = 10 μm; inset in (F) = 5 μm; insets in (I,J) = 2.5 μm.
Dopaminergic Regulation of Striatal Gαolf Protein Levels
To examine the dopaminergic regulation of Gαolf protein levels in the striatum, we performed a quantitative IHC using an anti-Gαolf antibody on striatal sections prepared from naïve control, PD, and PD-D mice. In low-magnification microscopic images, PD mice (Figure 3A) showed dramatic increases in Gαolf IR in the dorsal striatum on the 6-OHDA-lesioned side when compared to non-lesioned side. In contrast, PD-D mice (Figure 3B) only had a modest increase in striatal Gαolf IR on the 6-OHDA-lesioned side relative to non-lesioned side. Higher-magnification microscopic images of the DL striatum also show that, when compared to naïve controls (Figure 3C), there is a marked, but, slight increase in Gαolf IR in the 6-OHDA-lesioned striatal areas of PD (Figure 3D) and PD-D (Figure 3E) mice. As indicated in a previous report (Ruiz-DeDiego et al., 2015), it is likely that dopamine depletion increases Gαolf IR mainly in the matrix of the 6-OHDA-lesioned striatum, leading to a loss of the striosome-predominant pattern of Gαolf IR expression in PD mice. However, daily treatment with L-DOPA reverses the lesion-induced increase in Gαolf IR primarily in the matrix, leading to reappearance of the striosome-predominant pattern of Gαolf IR expression in PD-D mice. These visual impressions were confirmed by quantitative densitometry analyses of the DL striatum (Figures 3F–H), as follows. We found a significant and marked increase of 101% (P < 0.001, two-way ANOVA) in Gαolf IR levels in the 6-OHDA-lesioned striatum of PD, when compared to naïve controls. There was a 67% decrease (P < 0.01, two-way ANOVA) in Gαolf IR levels in the 6-OHDA-lesioned striatum of PD-D mice when compared to that of PD mice (Figure 3F; PD mice: non-lesion, 102 ± 17% and 6-OHDA-lesion, 201 ± 28%; PD-D mice: non-lesion, 103 ± 19% and 6-OHDA-lesion, 134 ± 16%; % of naïve control mice ± SEM; n = 15).
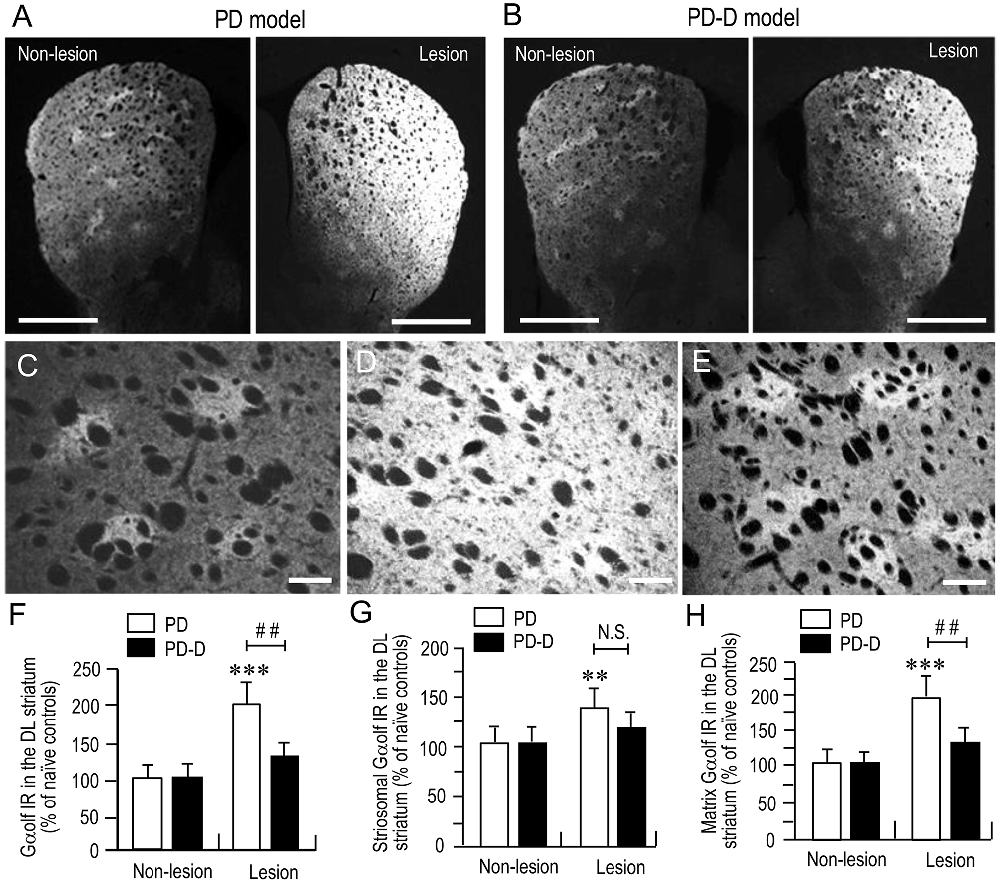
Figure 3. Dopaminergic regulation of striatal Gαolf levels. (A,B) Representative low-magnification microscopic images of striatal sections stained for Gαolf on the non-lesion and lesion sides from 6-OHDA-lesioned mice treated with daily injections of benserazide alone for 10 days (PD model; (A) and 6-OHDA-lesioned mice that received daily injections of benserazide and L-DOPA for 10 days and exhibited dyskinesia (PD-D model; B). (C–E) Representative higher-magnification microscopic images of the DL striatum stained for Gαolf from naïve control (C), PD (D) and PD-D (E) mice. (F–H) Optical density quantification of Gαolf IR in the DL striatum on the non-lesion and lesion sides from PD (n = 15) and PD-D (n = 15) mice. Data are expressed as percentage of naïve control mice (n = 15) and are means ± SEM. (F) Quantification of Gαolf IR in the DL regions in the striatum. ***P < 0.001 vs. naïve controls; ##P < 0.01 vs. PD; two-way analysis of variance (ANOVA) (F(1,56) = 75.5) followed by Bonferroni’s test. (G) Quantification of Gαolf IR in the striosome subfields in the DL striatum. **P < 0.01 vs. naïve controls; N.S. (not significant) vs. PD; two-way ANOVA (F(1,56) = 9.2) followed by Bonferroni’s test. (H) Quantification of Gαolf IR in the matrix subfields in the DL striatum. ***P < 0.001 vs. naïve controls; ##P < 0.01 vs. PD; two-way ANOVA (F(1,56) = 89.6) followed by Bonferroni’s test. Scale bars: (A,B) = 2 mm; (C–E) = 100 μm.
In the striatal compartments of the DL striatum, we found a significant increase of 38% (P < 0.01, two-way ANOVA) Gαolf IR levels in the striosomes of 6-OHDA-lesioned striatum of PD, when compared to naïve controls. There was no apparent difference (P > 0.05, two-way ANOVA) in striosomal levels of Gαolf IR in the 6-OHDA-lesioned striatum between PD and PD-D mice (Figure 3G; PD mice: non-lesion, 101 ± 22% and 6-OHDA-lesion, 138 ± 26%; PD-D mice: non-lesion, 102 ± 19% and 6-OHDA-lesion, 122 ± 20%; % of naïve control mice ± SEM; n = 15). We also found a significant increase of 96% (P < 0.001, two-way ANOVA) in matrix levels of Gαolf IR in the 6-OHDA-lesioned striatum of PD mice, when compared to those of naïve controls. There was a 66% decrease (P < 0.01, two-way ANOVA) in matrix levels of Gαolf IR in the 6-OHDA-lesioned striatum of PD-D mice when compared to those of PD mice (Figure 3H; PD mice: non-lesion, 99 ± 23% and 6-OHDA-lesion, 196 ± 24%; PD-D mice: non-lesion, 102 ± 12% and 6-OHDA-lesion, 130 ± 22%; % of naïve control mice ± SEM; n = 15). These findings indicate that dopamine depletion causes a dramatic increase in Gαolf levels in the DL striatum, particularly in the matrix. Daily exposure to L-DOPA induces a down-regulation of this lesion-induced increase in Gαolf expression.
Dopaminergic Regulation of Striatal Expression of D1R, A2AR, and D2R
To examine dopaminergic regulation of D1R, A2AR and D2R expression in the DL striatum, we performed quantitative IHC on sections prepared from 6-OHDA-lesioned striata of PD and PD-D mice (Figure 4). We observed no significant changes (P > 0.05, one-way ANOVA) in the expression levels of D1R IR in PD or PD-D mice when compared to naïve controls (Figures 4A,B; PD mice, 101 ± 21%; PD-D mice, 98 ± 17%; % of naïve control mice ± SEM; n = 15). The expression levels of A2AR IR in PD and PD-D mice were not significantly different (P > 0.05, one-way ANOVA) from those in naïve controls (Figures 4C,D; PD mice, 103 ± 15%; PD-D mice, 118 ± 13%; % of naïve control mice ± SEM; n = 15). We found a significant increase in the expression of D2R in PD (P < 0.01, one-way ANOVA), but not PD-D (P > 0.05, one-way ANOVA), mice when compared to naïve controls (Figures 4E,F; PD mice, 123 ± 15%; PD-D mice, 112 ± 22%; % of naïve control mice ± SEM; n = 15). These findings indicate that dopamine depletion causes a significant increase in striatal D2R expression, which is reversed by daily treatment with L-DOPA. In addition, dopamine depletion and L-DOPA replacement cause no significant changes in striatal expression of D1R and A2AR in the dopamine-denervated striatum.
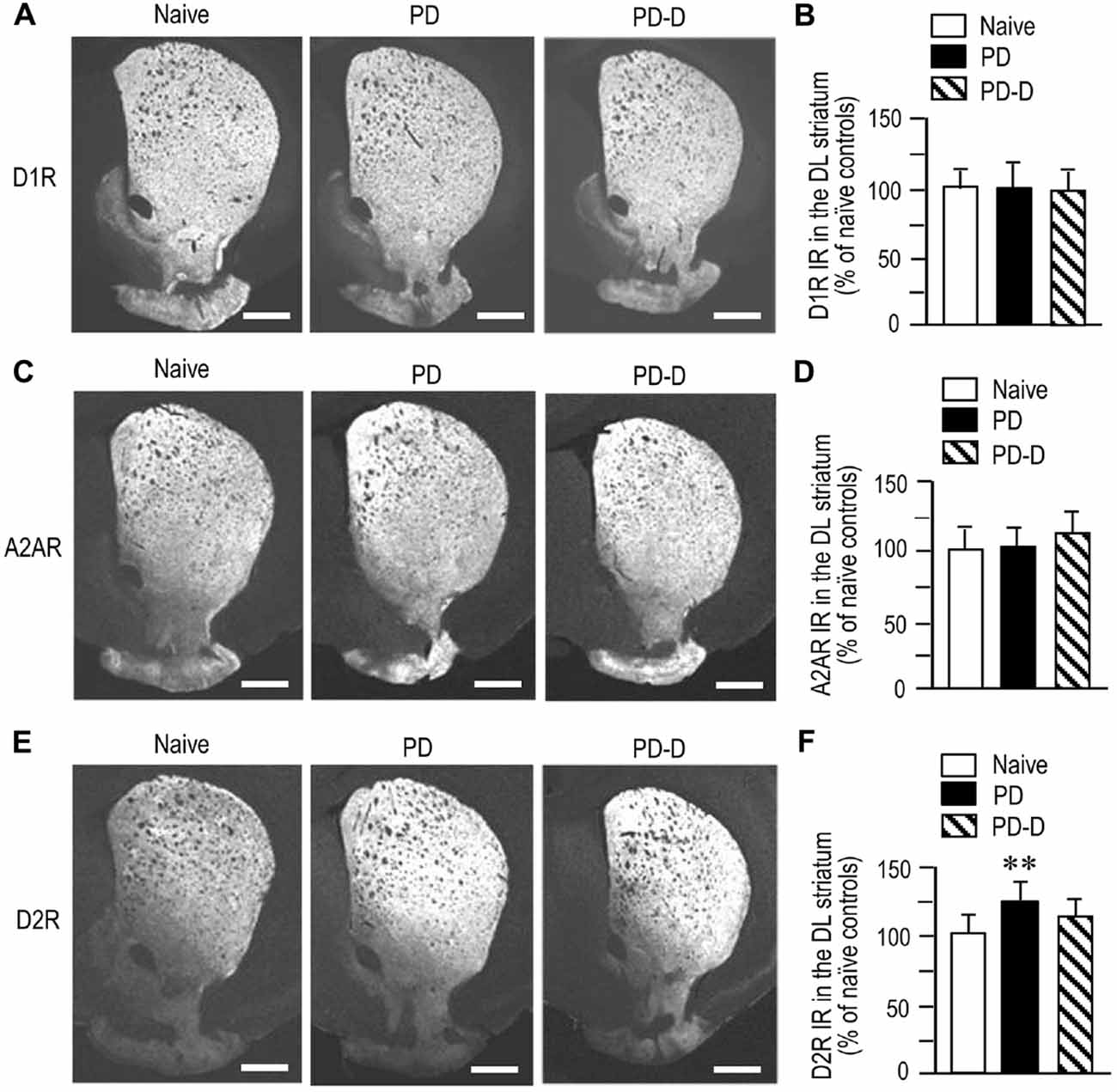
Figure 4. Dopaminergic regulation of striatal expression of D1R, A2AR and D2R. (A) Representative photomicrographs of striatal expression of D1R in normal (naïve controls) and lesioned hemispheres from 6-OHDA-lesioned mice treated with daily injections of benserazide alone for 10 days (PD model), and from 6-OHDA-lesioned mice that received daily injections of benserazide and L-DOPA for 10 days and exhibited dyskinesia (PD-D model). (B) Optical density quantification of D1R IR in the DL striatum from PD (n = 15) and PD-D (n = 15) mice. Data are expressed as percentage of naïve control mice (n = 15) and are means ± SEM. No significant changes in striatal levels of D1R IR in PD and PD-D mice were observed when compared to naïve controls; one-way ANOVA (F(2,42) = 0.0) followed by Bonferroni’s test. (C) Representative photomicrographs of striatal expression of A2AR from naïve control, PD and PD-D mice. (D) Optical density quantification of A2AR IR in the DL striatum from PD (n = 15) and PD-D (n = 15) mice. Data are expressed as percentage of levels in naïve control mice (n = 15) and are means ± SEM. No significant changes in striatal levels of A2AR IR in PD and PD-D mice were observed when compared to naïve controls; one-way ANOVA (F(2,42) = 1.2) followed by Bonferroni’s test. (E) Representative photomicrographs of striatal expression of D2R from naïve control, PD and PD-D mice. (F) Optical density quantification of D2R IR in the DL striatum from PD (n = 15) and PD-D (n = 15) mice. Data are expressed as percentage of levels in naïve control mice (n = 15) and are means ± SEM. **P < 0.01 vs. naïve controls; one-way ANOVA (F(2,42) = 16.9) followed by Bonferroni’s test. Scale bars: (A,C,E) = 1 mm.
Dopaminergic Regulation of Striatal Levels of PLA Signals for D1R-Gαolf
To examine the dopaminergic regulation of striatal levels of Gαolf protein in close proximity to D1R protein, we used a sensitive in situ PLA in sections prepared from 6-OHDA-lesioned striata from PD and PD-D mice (Figure 5). In low-magnification microscopic images, a marked and moderate increase in D1R-Gαolf PLA signals was observed in the dorsal striatum in PD and PD-D mice when compared to naïve controls (Figures 5A,B). Higher-magnification microscopic images of the DL striatum also show that compared to naïve controls (Figure 5C), there is a marked and moderate increase in the D1R-Gαolf PLA signals in 6-OHDA-lesioned striatal areas in PD (Figure 5D) and PD-D (Figure 5E) mice. Quantitative densitometry analyses of the DL striatum revealed increases of 92% (P < 0.001, one-way ANOVA) and 50% (P < 0.001, one-way ANOVA) in the D1R-Gαolf PLA signal in PD and PD-D mice, respectively, when compared to naïve controls. There was a decrease of 42% (P < 0.001, one-way ANOVA) in the D1R-Gαolf PLA signal in PD-D mice when compared to PD mice (Figure 5F; PD mice, 192 ± 25%; PD-D mice, 150 ± 21%; % of naïve control mice ± SEM; n = 10). These findings indicate that dopamine depletion causes a marked increase in striatal D1R-Gαolf PLA signal, which is downregulated by daily treatment with L-DOPA. However, there is a significant increase of striatal D1R-Gαolf PLA signal in PD-D mice compared to naïve controls.
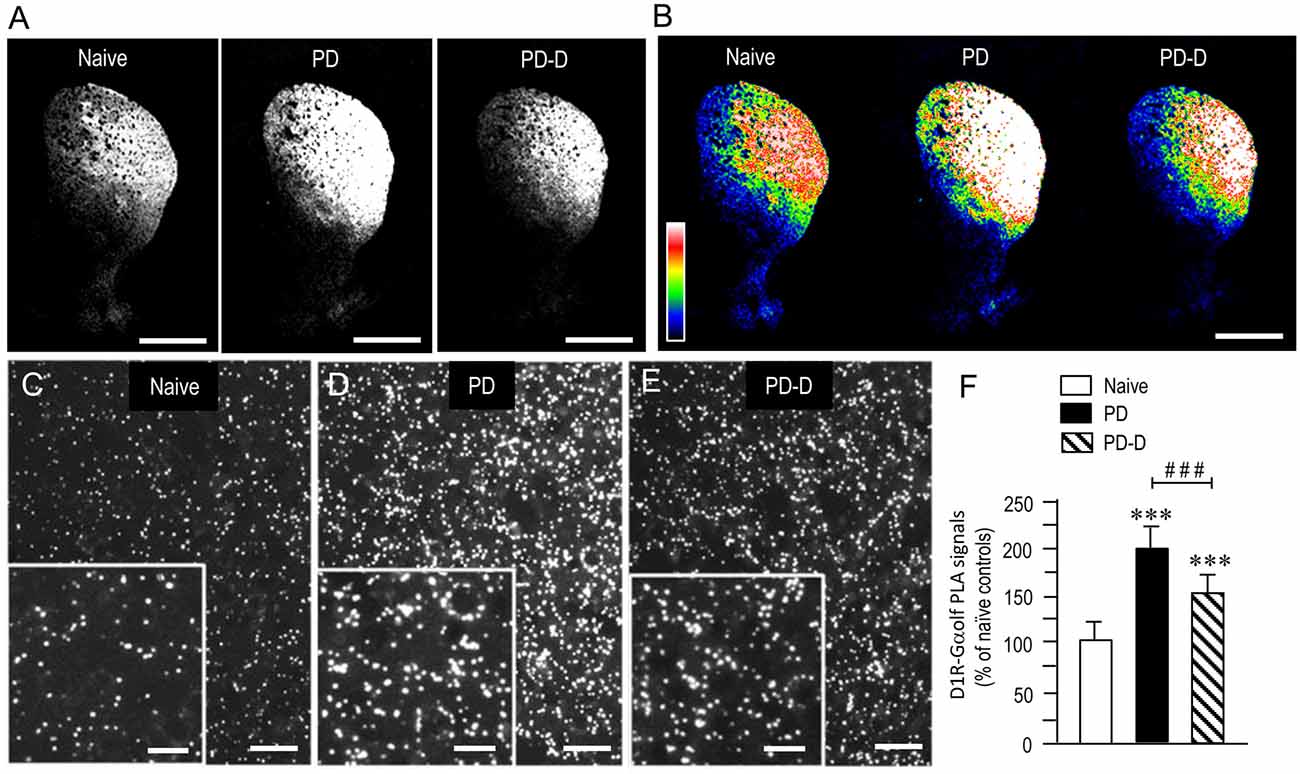
Figure 5. Dopaminergic regulation of striatal levels of Gαolf proteins in close proximity to D1R proteins. Dual-antigen recognition in situ PLA used to detect Gαolf proteins in proximity to D1R proteins (D1R-Gαolf) was carried out on normal hemispheres of naïve controls and on lesioned hemispheres from 6-OHDA-lesioned mice treated with daily injections of benserazide alone for 10 days (PD model) and from 6-OHDA-lesioned mice that received daily injections of benserazide and L-DOPA for 10 days and exhibited dyskinesia (PD-D model). (A,B) Representative photomicrographs of striatal expression of D1R-Gαolf PLA signals in normal and lesioned hemispheres from PD and PD-D mice (A), and their graded color-converted images (B). (C–E) Representative photomicrographs of the DL striatum stained with the in situ PLA for D1R-Gαolf from naïve control (C), PD (D) and PD-D (E) mice. Their higher-magnification images are also shown in the insets in (C–E). (F) Optical density quantification of D1R-Gαolf PLA signals in the DL striatum from PD (n = 10) and PD-D (n = 10) mice. Data are expressed as percentage of naïve control mice (n = 10) and are means ± SEM. ***P < 0.001 vs. normal controls; ###P < 0.001 vs. PD; one-way ANOVA (F(2,27) = 107.2) followed by Bonferroni’s test. Scale bars: (A,B) = 2 mm; (C–E) = 25 μm; insets in (C–E) = 10 μm.
Dopaminergic Regulation of Striatal Levels of PLA Signals for A2AR-Gαolf
To examine the dopaminergic regulation of striatal levels of Gαolf protein in close proximity to A2AR protein, we used a sensitive in situ PLA in sections prepared from 6-OHDA-lesioned striata from PD and PD-D mice (Figure 6). Notably, low-magnification microscopic images show an apparent decrease in the A2AR-Gαolf PLA signal in the DL striatum of PD-D mice when compared to both naïve control and PD mice (Figures 6A,B). Higher-magnification images also show the localization patterns of A2AR-Gαolf PLA signals in the DL striatum of naïve control (Figure 6C), PD (Figure 6D), and PD-D (Figure 6E) mice. Quantitative densitometry analyses of the DL striatum revealed decreases of 41% (P < 0.001, one-way ANOVA) and 45% (P < 0.001, one-way ANOVA) in A2AR-Gαolf PLA signal levels in PD-D mice, when compared to naïve controls and PD mice, respectively (Figure 6F; PD mice, 104 ± 24%; PD-D mice, 59 ± 21%; % of naïve control mice ± SEM; n = 10). These findings indicate that L-DOPA replacement, but not dopamine depletion, causes a significant decrease in the striatal A2AR-Gαolf PLA signal in the dopamine-denervated striatum.
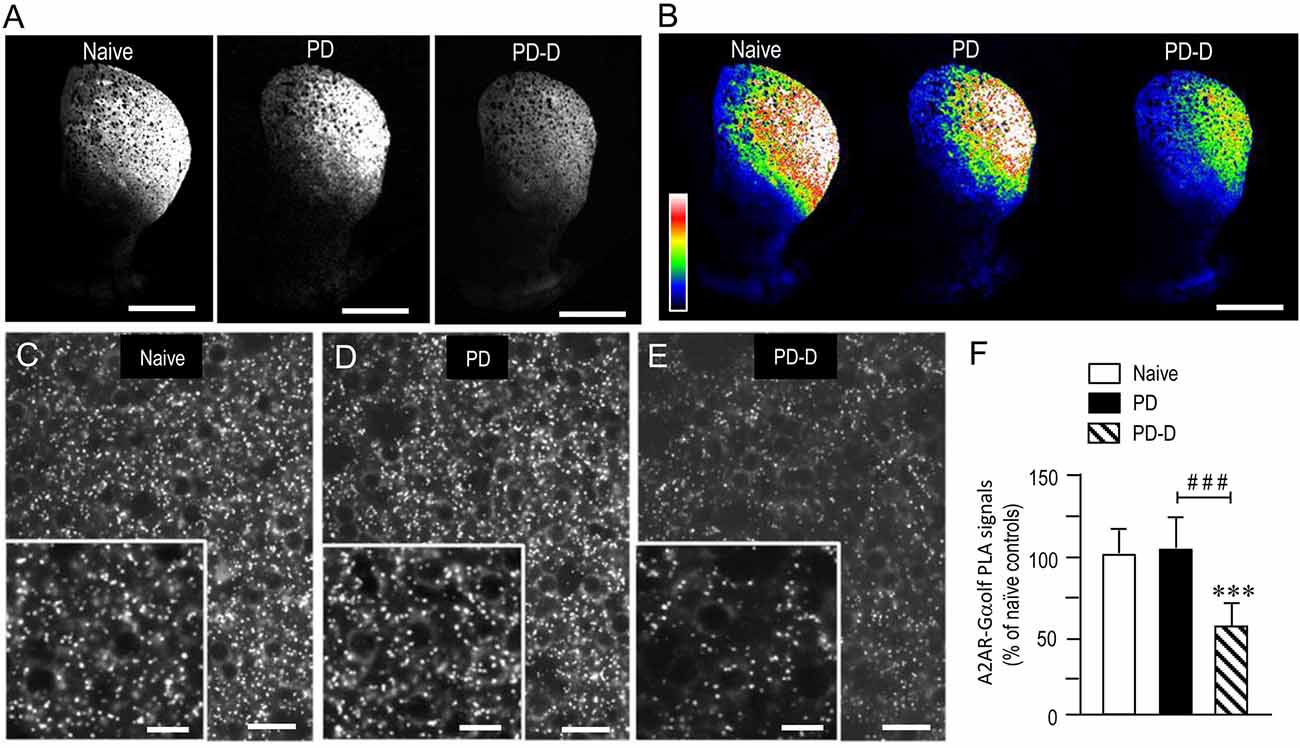
Figure 6. Dopaminergic regulation of striatal levels of Gαolf proteins in close proximity to A2AR proteins. Dual-antigen recognition in situ PLA used to detect Gαolf proteins in proximity to A2AR proteins (A2AR-Gαolf) was carried out on normal hemispheres of naïve controls and on lesioned hemispheres from 6-OHDA-lesioned mice treated with daily injections of benserazide alone for 10 days (PD model) and from 6-OHDA-lesioned mice that received daily injections of benserazide and L-DOPA for 10 days and exhibited dyskinesia (PD-D model). (A,B) Representative photomicrographs of striatal expression of A2AR-Gαolf PLA signals in normal and lesioned hemispheres from PD and PD-D mice (A), and their graded color-converted images (B). (C–E) Representative photomicrographs of the DL striatum stained with the in situ PLA for A2AR-Gαolf from naïve control (C), PD (D) and PD-D (E) mice. Their higher-magnification images are also shown in the insets in (C–E). (F) Optical density quantification of A2AR-Gαolf PLA signals in the DL striatum from PD (n = 10) and PD-D (n = 10) mice. Data are expressed as percentage of naïve control mice (n = 10) and are means ± SEM. ***P < 0.001 vs. naive controls; ###P < 0.001 vs. PD; one-way ANOVA (F(2,27) = 72.6) followed by Bonferroni’s test. Scale bars: (A,B) = 2 mm; (C–E) = 25 μm; insets in (C–E) = 10 μm.
Differences in Striatal Responsiveness to Dopamine Stimulation between PD and PD-D Mice
To assess changes in striatal responsiveness to dopamine stimulation in PD and PD-D mice, we performed IHC using an antibody against c-Fos, which is known to be induced in the striatum and globus pallidus following the stimulation of D1Rs and D2Rs (Marshall et al., 1993; LaHoste and Marshall, 1994). We prepared striatal sections from PD and PD-D mice that received injections of L-DOPA (20 mg/kg) and benserazide-HCl (12 mg/kg) 2 h before sacrifice on day 11 (see Figure 1A). Microscopic images of the DL striatum stained for c-Fos from naïve control, PD and PD-D mice are shown in Figure 7A. Compared to naïve controls that also received the injections of L-DOPA (20 mg/kg) and benserazide-HCl (12 mg/kg) 2 h before sacrifice, we found a marked increase in the densities of c-Fos-positive (c-Fos+) nuclei in the 6-OHDA-lesioned striatum in both PD and PD-D mice. Quantitative densitometry analyses also showed a marked increase (P < 0.001, two-way ANOVA) in the density of c-Fos+ nuclei in the 6-OHDA-lesioned striatum in both PD and PD-D mice when compared to naïve controls. However, there was a decrease of ~40% (P < 0.001, two-way ANOVA) in the density of c-Fos+ nuclei in the 6-OHDA-lesioned striatum of PD-D mice when compared to PD mice (Figure 7B; naïve controls: 20 ± 12; PD mice: non-lesion, 31 ± 10 and 6-OHDA-lesion, 475 ± 55; PD-D mice: non-lesion, 29 ± 12 and 6-OHDA-lesion, 292 ± 49; means ± SEM; n = 10). Microscopic images of the globus pallidus stained for c-Fos obtained from naïve control, PD, and PD-D mice are shown in Figure 7C. Compared to naïve controls, we found increased densities of c-Fos+ nuclei in the globus pallidus on the lesioned sides in both PD and PD-D mice. Quantitative densitometry analyses also indicated a significant increase (P < 0.001, two-way ANOVA) in the density of c-Fos+ nuclei in the globus pallidus on the lesioned sides in both PD and PD-D mice when compared to naïve controls. Importantly, we found that there was an increase of ~140% (P < 0.01, two-way ANOVA) in the density of c-Fos+ nuclei in the globus pallidus on the lesioned side in PD-D mice when compared to PD mice (Figure 7D; naïve controls: 4 ± 2; PD mice: non-lesion, 5 ± 3 and 6-OHDA-lesion, 38 ± 8; PD-D mice: non-lesion, 8 ± 7 and 6-OHDA-lesion, 92 ± 11; means ± SEM; n = 10). These findings indicate that dopamine depletion causes a marked increase in the responsiveness of striatal D1-cells to dopamine stimulation, which is downregulated by daily treatments with L-DOPA. Given the changes in striatal D2R expression in PD and PD-D mice (see above), it is likely that in the dopamine-denervated striatum, dopamine depletion may cause increased striatal D2R expression, which then enhances the responsiveness of D2-cells to dopamine stimulation (Cai et al., 2002). Notably, L-DOPA replacement could induce a further increase in the responsiveness of D2-cells to dopamine stimulation despite no obvious increase in striatal D2R expression in the dopamine-denervated striatum.
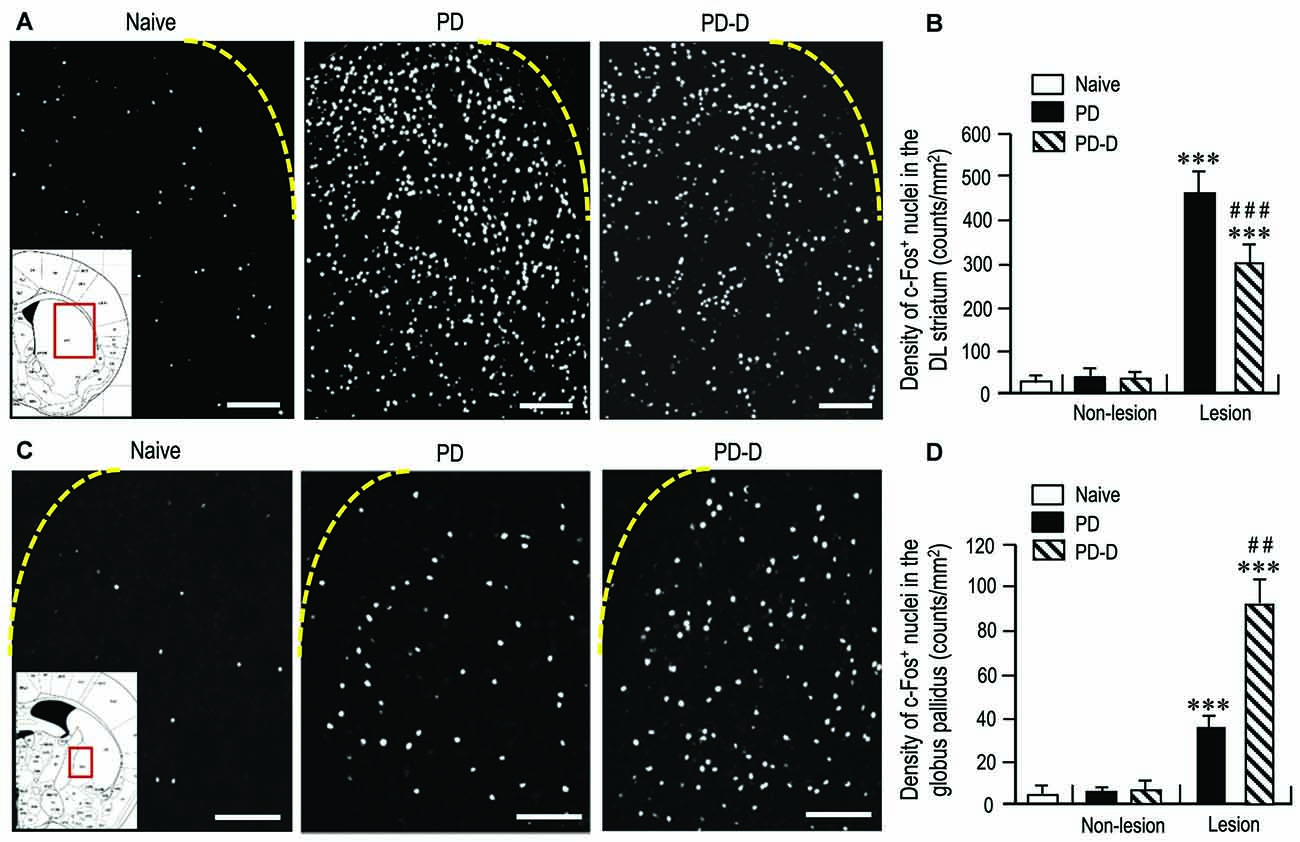
Figure 7. Effects of dopamine stimulation on striatal and pallidal c-Fos expression in 6-OHDA-lesioned mice. An immunohistochemical study with anti-c-Fos antibody was carried out on the striatal sections from PD and PD-D mice, which received injections of L-DOPA (20 mg/kg) and benserazide-HCl (12 mg/kg) 2 h before sacrifice on day 11 (see Figure 1A), and those from naïve controls that also received the injections of L-DOPA (20 mg/kg) and benserazide-HCl (12 mg/kg) 2 h before sacrifice. The density measurements were performed by counting the numbers of c-Fos-positive (c-Fos+) nuclei in a 0.5 mm × 0.5 mm field in the striatum and globus pallidus from each animal. (A) Representative photomicrographs of the DL striatum stained for c-Fos in naïve control, PD and PD-D mice. The inset (red open box) in the naïve control is the corresponding figure from the atlas of Paxinos and Franklin (2001) to show the striatal area that were analyzed in all naïve control, PD and PD-D mice. (B) Density quantification of c-Fos+ nuclei in the DL striatum from naïve controls (n = 10), and of those on non-lesion and lesion sides in PD (n = 10) and PD-D (n = 10) mice. Data are expressed as means ± SEM. ***P < 0.001 vs. naïve controls; ###P < 0.001 vs. PD; two-way ANOVA (F(1,36) = 140.9) followed by Bonferroni’s test. (C) Representative photomicrographs of the globus pallidus stained for c-Fos in naïve control, PD and PD-D mice. The inset (red open box) in the naïve control is the corresponding figure from the atlas of Paxinos and Franklin (2001) to show the pallidal area that were analyzed in all naïve control, PD and PD-D mice. (D) Density quantification of c-Fos+ nuclei in the globus pallidus from naïve controls (n = 10), and of those on non-lesion and lesion sides in PD (n = 10) and PD-D (n = 10) mice. Data are expressed as means ± SEM. ***P < 0.001 vs. naïve controls; ##P < 0.01 vs. PD; two-way ANOVA (F(1,36) = 80.8) followed by Bonferroni’s test. Scale bars: (A) = 200 μm; (C) = 100 μm.
Discussion
Here we used IHC and in situ PLA to determine the region- and cell-type- specific distributions of Gαolf proteins in the mouse striatum. Using a mouse model of hemiparkinsonism induced by 6-OHDA, we also found that daily pulsatile administration of L-DOPA might induce usage-dependent changes in Gαolf expression not only in D1-cells, but also in D2-cells in the dopamine-depleted striatum (see Figure 8). This raises the possibility that LID might result from reduced A2AR/Gαolf/cAMP signal levels in D2-cells, which may be caused by intermittent and pulsatile activation of postsynaptic D1Rs in the striatum. Our results support and provide new insights into the hypothesis that LID is associated with a decrease in activity of “indirect” striatopallidal pathway (Crossman, 1990; DeLong, 1990; Brotchie, 2005; Guridi et al., 2012).
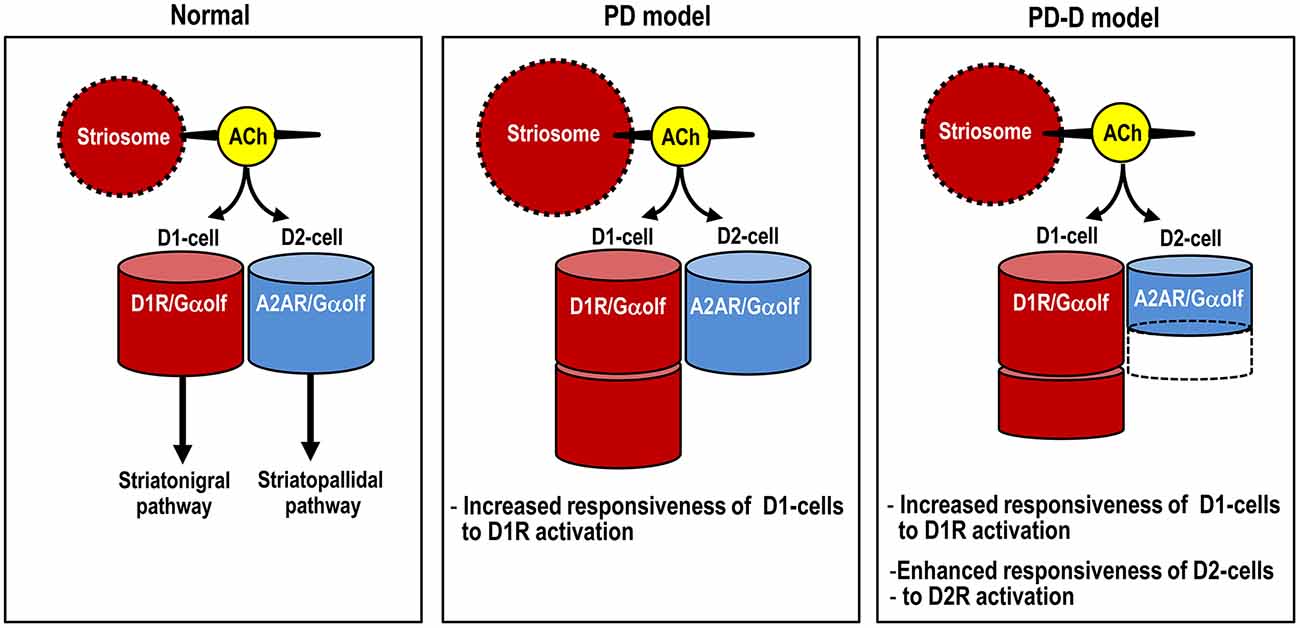
Figure 8. Proposed diagram for dopaminergic regulation of Gαolf levels that determine responsiveness to dopamine stimulation in striatal D1- and D2-cells. The heights of the red and blue columns indicate the abundance of Gαolf proteins in D1-cells and D2-cells, respectively. In PD mice, D1-cells might exhibit the dopamine D1R hypersensitivity caused by a dramatic increase in their Gαolf levels, while D2-cells might show no apparent changes in their Gαolf levels. In PD-D mice, D1-cells might show an increase in their Gαolf levels, while D2-cells might show a decrease in their Gαolf levels, which might result in an enhanced responsiveness to D2R activation. Abbreviations: PD, Parkinson’s disease: PD-D, PD with dyskinesia; ACh, acetylcholine; D1-cell, dopamine D1 receptor-expressing striatonigral medium spiny neuron; D2-cell, dopamine D2 receptor-expressing striatopallidal medium spiny neuron; D1R, dopamine D1 receptor; D2R, dopamine D2 receptor; Gαolf, olfactory type G-protein α subunit.
Strategic Localization of Gαolf Proteins in the Striatum
We used IHC to demonstrate that Gαolf IR is highly concentrated in the DL striatum, which corresponds to the motor-sensory territory in rodents and is analogous to the putamen in primates (Graybiel, 2008). This implies that Gαolf may have a unique position in regulating the activities of the cortico-thalamo-basal ganglia circuit involved in motor functions, i.e., the motor loop (Alexander and Crutcher, 1990), at the striatal level. Although a previous study revealed no obvious compartmental difference in Gαolf mRNA expression throughout striatal development in rats (Sakagami et al., 1995), we observed differential concentrations of Gαolf IR in the striosome and matrix compartments, with higher densities of Gαolf IR in the striosomes relative to the matrix. This finding suggests that Gαolf may be a key molecule for controlling differential responses of striosome-matrix systems to D1R activation in adult mice. There is evidence that in experimental animal models with 6-OHDA-lesions (Hervé et al., 1993; Corvol et al., 2004; Alcacer et al., 2012; Ruiz-DeDiego et al., 2015) or in those with a total absence of D1Rs due to D1R gene targeting (Hervé et al., 2001), the upregulation of Gαolf levels in the striatum is not accompanied by a parallel increase in Gαolf mRNA expression. Thus, homeostatic regulation of striatal Gαolf protein levels is thought to occur via post-translational mechanisms, wherein the altered expression of Gαolf protein depends directly on its rate of usage (Hervé, 2011). We suggest that when compared to the matrix, the striosomes might have the lower levels of D1R/Gαolf stimulation, which may then lower the Gαolf degradation rate and lead to accumulation of the protein. Our assumption is supported by the present finding that changes in striatal Gαolf IR expression were primarily found in the matrix in both PD and PD-D mice.
In this study, we first used highly sensitive dual-antigen recognition in situ PLA using a combination of the Brightfield Duolink PLA kit reagents and the TSA system (see “Materials and Methods” Section). This dual-antigen recognition PLA technique allowed us to obtain specific and efficient fluorescent signals showing Gαolf protein in close proximity to D1R or A2AR protein in the striatum. However, we cannot say that all the PLA signals detected here resulted from the direct interaction (or actual coupling) of Gαolf protein with D1R or A2AR protein. Borroto-Escuela et al. (2013) have shown that PLA can indicate a close proximity between two proteins, which is not always a reflection of direct interaction. This is because in situ PLA signals can be detected when two protein epitopes are in close proximity with ranges of 10–30 nm or more. In addition, although the precise mechanisms by which Gαolf protein interact with D1R or A2AR protein remains unclear, it was also noted that in striatal membrane, the content in Gαolf protein would be almost one to two orders of magnitude higher than that in D1R or A2AR (Hervé, 2011).
Striatal Gαolf as a Determinant of the Increased Responsiveness of D1-cells to Dopamine Stimulation in LID
As shown in previous studies (Alcacer et al., 2012; Ruiz-DeDiego et al., 2015), we found a marked increase in striatal Gαolf protein levels in PD mice with 6-OHDA lesions. This is in line with evidence that dopamine depletion may lead to up-regulation of Gαolf protein expression in the rat striatum (Hervé et al., 1993; Marcotte et al., 1994; Penit-Soria et al., 1997; Corvol et al., 2004; Rangel-Barajas et al., 2011) and in the putamen in patients with PD (Corvol et al., 2004). Given the evidence that striatal levels of D1R (Shinotoh et al., 1993; Turjanski et al., 1997: Hurley et al., 2001) and other major mediators of D1R signaling (Girault et al., 1989; Nishino et al., 1993) are unchanged in PD patients, the dramatic increase in striatal Gαolf protein level may be a key event in the D1R hypersensitivity that develops in PD (Alcacer et al., 2012). In support of this notion, we detected no obvious changes in striatal D1R expression in PD mice.
Previous data have suggested that the up-regulation of Gαolf protein levels in the dopamine-depleted striatum is post-translational (Hervé et al., 1993; Ruiz-DeDiego et al., 2015) and results from the disuse of the D1Rs (Hervé et al., 2001). Indeed, daily administration of L-DOPA for 10 days resulted in a down-regulation of the increased Gαolf protein levels in the 6-OHDA-lesioned striatum in PD-D mice. However, we also found a significant increase in striatal Gαolf levels in PD-D mice when compared to naïve controls. In agreement with the changes in striatal Gαolf levels in PD and PD-D mice, in situ PLA also revealed that striatal D1R-Gαolf PLA signals were dramatically increased in PD mice and moderately increased in PD-D mice. These findings imply an increased responsiveness of D1-cells to D1R activation in PD-D mice, although this responsiveness is lower than that found in PD mice. Our assumption is also supported by the fact that, compared to naïve controls, a significant increase in the number of striatal c-Fos+ nuclei consequent to L-DOPA administration was evident in PD-D mice, although this increase was more pronounced in PD mice. Since Gαolf represents the rate-limiting factor in D1R-mediated cAMP production in D1-cells, these findings suggest that striatal Gαolf level acts as a determinant for the increased responsiveness of D1-cells to dopamine stimulation in LID (see Figure 8).
Striatal Gαolf as a Determinant of the Increased Responsiveness of D2-cells to Dopamine Stimulation in LID
It has been postulated that repeated exposure to dopaminergic agents leads to increased sensitivity of D2-cells to D2R activation in the dopamine-depleted striatum in experimental animals (Engber et al., 1989; Asin et al., 1995; Kashihara et al., 2000). However, no obvious increase in striatal D2R expression has been observed in PD patients treated with dopaminergic drugs (Rinne et al., 1981; Guttman and Seeman, 1985; Antonini et al., 1997; Thobois et al., 2004). In agreement with this notion, we found that pallidal c-Fos induction consequent to L-DOPA administration was more marked in PD-D mice compared to PD mice. On the other hand, there was increased expression of striatal D2Rs in PD mice, but not in PD-D mice. This indicates that the repeated administration of L-DOPA results in an increased responsiveness of D2-cells to striatal D2R activation in the dopamine-denervated striatum, and suggests that this phenomenon might underlie LID. Using an in situ PLA, we found that A2AR-Gαolf PLA signals were markedly reduced along with Gαolf protein levels in the 6-OHDA-lesioned striatum of PD-D mice. This novel finding indicates that as in D1-cells, repeated exposure to L-DOPA causes down-regulation of Gαolf protein levels in D2-cells in the dopamine-depleted striatum. This then leads to the facilitation of the effects of dopamine on D2-cells by reducing A2AR/Gαolf signaling-mediated cAMP production (see Figure 8). This may be the reason that PD-D mice display an increased responsiveness of D2-cells to dopamine stimulation. However, the mechanism by which repeated and pulsatile injections of L-DOPA causes a decrease in A2AR/Gαolf PLA signals in PD-D remains a matter of speculation, as follows.
A2AR usage by endogenous adenosine results in a basal rate of Gαolf degradation (Hervé et al., 2001). It has been shown that in experimental animals with 6-OHDA-lesions, chronic (or persistent) dopamine depletion caused no significant changes (Ballarin et al., 1987; Herrera-Marschitz et al., 1994; Nomoto et al., 2000) or slight decrease (Pinna et al., 2002) in the extracellular adenosine levels in the striatum. In accordance with these findings, our present results also showed no significant changes in striatal levels of A2AR-Gαolf PLA signals in PD mice. Thus, we suggest that chronic dopamine depletion per se might cause no obvious changes in A2AR/Gαolf signaling activities that depend on the endogenous adenosine levels in striatal D2-cells. However, it is known that endogenous levels of adenosine are increased in response to the activation of N-methyl-D-aspartate (NMDA) receptors (Delaney and Geiger, 1998; Delaney et al., 1998), which can be facilitated by D1R stimulation (Cepeda and Levine, 2012; Morigaki and Goto, 2015), in the striatum. A landmark report has shown that in the rat striatum, transient (pulsatile) stimulation of D1Rs facilitates the NMDA receptor-dependent increase in extracellular adenosine levels (Harvey and Lacey, 1997). These findings suggest that in 6-OHDA-lesioned mice with D1R hypersensitivity, repeated exposure to L-DOPA may lead to a transient activation of D1Rs, which then enhances the NMDA receptor-dependent increase in adenosine release in the dopamine-denervated striatum. Moreover, Nash and Brotchie (2000) have shown that in striatal slices prepared from rats with 6-OHDA lesions, NMDA receptor activation could cause a marked increase in adenosine release and, thereby, indirectly stimulate A2ARs. Taken together, we speculate that in the 6-OHDA-lesioned striatum of PD-D mice, decreased Gαolf levels in D2-cells might be due to increased extracellular adenosine levels caused by the daily pulsatile activation of striatal D1Rs. If our assumption is correct, striatal D1R signals might contribute to regulation of the Gαolf protein levels in not only D1-cells but also D2-cells in the dopamine-depleted striatum.
Because adenosine/A2AR signaling functionally opposes the actions of D2Rs on D2-cells by its ability to increase the A2AR/Gαolf-dependent cAMP production, it has so far been suggested that A2AR antagonism may boost the anti-parkinsonian action of D2R agonists in treating PD symptoms (Jenner, 2003; Schwarzschild et al., 2006; Fuxe et al., 2007; Huot et al., 2013). In addition, based on the evidence that striatal A2AR expression might be increased in PD patients with dyskinesia (Calon et al., 2004; Ramlackhansingh et al., 2011) and in dyskinetic animal models of PD (Jenner et al., 2009), it has also been suggested that adenosine A2A sites might be a potential pharmacologic target for reducing LIDs (Jenner et al., 2009; Ramlackhansingh et al., 2011; Huot et al., 2013; Kanda and Uchida, 2014). In Japan, istradefylline, an A2AR antagonist, is currently used in clinics for treating PD patients (Kondo and Mizuno, 2015). The drug has shown to improve “off” time in patients with advanced PD, but has not shown anti-LID effects in the absence of a reduction in dopaminergic drug dosage. Adjunct use of istradefylline often causes dyskinetic symptoms as a major adverse effect (Kondo and Mizuno, 2015). Considering usage-dependent Gαolf degradation through adenosine/A2AR, we assume that in PD patients treated with L-DOPA, adenosine/A2AR antagonism might be effective in reducing the “priming” of LID. However, once LID is established, adenosine/A2AR antagonism might exacerbate dyskinetic symptoms. Our assumption may corroborate the notion that A2AR activation might be required for dyskinesia “priming” mechanism (Brotchie, 2005).
Conclusion
Because Gαolf protein level serves as a determinant of cAMP signal-dependent activity in both D1-cells and D2-cells in the striatum, Gαolf may represent an ideal target for the modulation of striatal functions under physiological and pathological conditions. Dysregulation of Gαolf expression has been associated with the pathophysiology of several brain disorders (Hervé, 2011). Of our particular interest is that the GNAL gene, which encodes Gαolf, is a causative gene in primary (torsion) dystonia (Fuchs et al., 2013). This is direct evidence that Gαolf plays a pivotal role in the “motor loop” of the cortico-basal ganglia circuits. Under parkinsonian conditions, dopamine depletion results in a crucial D1R hypersensitivity in the striatum, which leads to the beneficial effects of L-DOPA in PD patients, but also generates LID. In this study, we found that in the 6-OHDA-lesioned striatum of PD mice, daily pulsatile administrations of L-DOPA may cause usage-induced changes in striatal Gαolf levels, leading to increased responsiveness to dopamine stimulation in both D1-cells and D2-cells. Thus we suggest that L-DOPA-induced changes in Gαolf levels in the dopamine-depleted striatum may be a key event in LID development.
Author Contributions
SG conceived and designed the experiments. RM, SO and SG performed the experiments; analyzed the data; contributed reagents/materials/analysis tools. SG wrote the manuscript.
Conflict of Interest Statement
The authors declare that the research was conducted in the absence of any commercial or financial relationships that could be construed as a potential conflict of interest.
Acknowledgments
This work was supported in part by grants from the Ministry of Education, Culture, Sports, Science and Technology of Japan (grants-in-aid for Scientific Research no. 24390223, 26461272, 26430054 and 16K10788) and Japan Agency for Medical Research and Development (AMED; no. 16ek0109182h0001).
References
Ahlskog, J. E., and Muenter, M. D. (2001). Frequency of levodopa-related dyskinesias and motor fluctuations as estimated from the cumulative literature. Mov. Disord. 16, 448–458. doi: 10.1002/mds.1090
Alcacer, C., Santini, E., Valjent, E., Gaven, F., Girault, J.-A., and Hervé, D. (2012). Gαolf mutation allows parsing the role of cAMP-dependent and extracellular signal-regulated kinase-dependent signaling in L-3,4,-dihydroxyphenylalanine-induced dyskinesia. J. Neurosci. 32, 5900–5910. doi: 10.1523/JNEUROSCI.0837-12.2012
Alexander, G. E., and Crutcher, M. D. (1990). Functional architecture of basal ganglia circuits: neural substrates of parallel processing. Trends Neurosci. 13, 266–271. doi: 10.1016/0166-2236(90)90107-l
Antonini, A., Schwarz, J., Oertel, W. H., Pogarell, O., and Leenders, K. L. (1997). Long-term changes of striatal dopamine D2 receptors in patients with Parkinson’s disease: a study with positron emission tomography and [11C] raclopride. Mov. Disord. 12, 33–38. doi: 10.1002/mds.870120107
Asin, K. E., Bednarz, L., Nikkel, A., and Perner, R. (1995). Rotation and striatal c-fos expression after repeated, daily treatment with selective dopamine receptor agonists and levodopa. J. Pharmacol. Exp. Ther. 273, 1483–1490.
Ballarin, M., Herrera-Marschitz, M., Casas, M., and Ungerstedt, U. (1987). Striatal adenosine levels measured ‘in vivo’ by microdialysis in rats with unilateral dopamine denervation. Neurosci. Lett. 83, 338–344. doi: 10.1016/0304-3940(87)90111-x
Bastide, M. F., Meissner, W. G., Picconi, B., Fasano, S., Fernagut, P. O., Feyder, M., et al. (2015). Pathophysiology of L-dopa-induced motor and non-motor complications in Parkinson’s disease. Prog. Neurobiol. 132, 96–168. doi: 10.1016/j.pneurobio.2015.07.002
Borroto-Escuela, D. O., Romero-Fernandez, W., Garriga, P., Ciruela, F., Narvaez, M., Tarakanov, A. O., et al. (2013). G protein-coupled receptor heterodimerization in the brain. Methods Enzymol. 521, 281–294. doi: 10.1016/B978-0-12-391862-8.00015-6
Brotchie, J. M. (2005). Nondopaminergic mechanisms in levodopa-induced dyskinesia. Mov. Disord. 20, 919–931. doi: 10.1002/mds.20612
Cai, G., Wang, H.-Y., and Friedman, E. (2002). Increased dopamine receptor signaling and dopamine receptor-G protein coupling in denervated striatum. J. Pharmacol. Exp. Ther. 302, 1105–1112. doi: 10.1124/jpet.102.036673
Calabresi, P., Di Filippo, M., Ghiglieri, V., Tambasco, N., and Picconi, B. (2010). Levodopa-induced dyskinesias in patients with Parkinson’s disease: filling the bench-to-bedside gap. Lancet Neurol. 9, 1106–1117. doi: 10.1016/s1474-4422(10)70218-0
Calabresi, P., Pisani, A., Rothwell, J., Ghiglieri, V., Obeso, J. A., and Picconi, B. (2016). Hyperkinetic disorders and loss of synaptic downscaling. Nat. Neurosci. 19, 868–875. doi: 10.1038/nn.4306
Calon, F., Dridi, M., Hornykiewicz, O., Bédard, P. J., Rajput, A. H., and Di Paolo, T. (2004). Increased adenosine A2A receptors in the brain of Parkinson’s disease patients with dyskinesias. Brain 127, 1075–1084. doi: 10.1093/brain/awh128
Cenci, M. A., Lee, C. S., and Björklund, A. (1998). L-DOPA-induced dyskinesia in the rat is associated with striatal overexpression of prodynorphin- and glutamic acid decarboxylase mRNA. Eur. J. Neurosci. 10, 2694–2706. doi: 10.1046/j.1460-9568.1998.00285.x
Cenci, M. A., Tranberg, A., Andersson, M., and Hilbertson, A. (1999). Changes in the regional and compartmental distribution of FosB- and JunB-like immunoreactivity induced in the dopamine-denervated rat striatum by acute or chronic L-dopa treatment. Neuroscience 94, 515–527. doi: 10.1016/s0306-4522(99)00294-8
Cepeda, C., and Levine, M. S. (2012). Dopamine-NMDA receptor interations: twenty years later. Dev. Neurosci. 34, 2–4. doi: 10.1159/000338590
Corvol, J. C., Muriel, M.-P., Valjent, E., Féger, J., Hanoun, N., Girault, J.-A., et al. (2004). Persistent increase in olfactory type G-protein α subunit levels may underlie D1 receptor functional hypersensitivity in Parkinson’s disease. J. Neurosci. 24, 7007–7014. doi: 10.1523/JNEUROSCI.0676-04.2004
Corvol, J. C., Studler, J. M., Schonn, J. S., Girault, J. A., and Hervé, D. (2001). Gαolf is necessary for coupling D1 and A2a receptors to adenylyl cyclase in the striatum. J. Neurochem. 76, 1585–1588. doi: 10.1046/j.1471-4159.2001.00201.x
Corvol, J. C., Valjent, E., Pascoli, V., Robin, A., Stipanovich, A., Luedtke, R. R., et al. (2007). Quantitative changes in Gαolf protein levels, but not D1 receptor, alter specifically acute responses to psychostimulants. Neuropsychopharmacology 32, 1109–1121. doi: 10.1038/sj.npp.1301230
Crittenden, J. R., and Graybiel, A. M. (2011). Basal ganglia disorders associated with imbalances in the striatal striosome and matrix compartments. Front. Neuroanat. 5:59. doi: 10.3389/fnana.2011.00059
Crossman, A. R. (1990). A hypothesis on the pathophysiological mechanisms that underlie levodopa- or dopamine agonist-induced dyskinesia in Parkinson’s disease: implications for future strategies in treatment. Mov. Disord. 5, 100–108. doi: 10.1002/mds.870050203
Darmopil, S., Martín, A. B., De Diego, I. R., Ares, S., and Moratalla, R. (2009). Genetic inactivation of dopamine D1 but not D2 receptors inhibits L-DOPA-induced dyskinesia and histone activation. Biol. Psychiatry 66, 603–613. doi: 10.1016/j.biopsych.2009.04.025
Delaney, S. M., and Geiger, J. D. (1998). Levels of endogenous adenosine in rat striatum. II. Regulation of basal and N-methyl-D-aspartate-induced levels by inhibitors of adenosine transport and metabolism. J. Pharmacol. Exp. Ther. 285, 568–572.
Delaney, S. M., Shepel, P. N., and Geiger, J. D. (1998). Levels of endogenous adenosine in rat striatum I. Regulation by ionotropic glutamate receptors, nitric oxide and free radicals. J. Pharmacol. Exp. Ther. 285, 561–567.
DeLong, M. R. (1990). Primate models of movement disorders of basal ganglia origin. Trends Neurosci. 13, 281–285. doi: 10.1016/0166-2236(90)90110-v
Ding, Y., Won, L., Britt, J. P., Lim, S. A., McGehee, D. S., and Kang, U. J. (2011). Enhanced striatal cholinergic neuronal activity mediates L-DOPA-induced dyskinesia in parkinsonian mice. Proc. Natl. Acad. Sci. U S A 108, 840–845. doi: 10.1073/pnas.1006511108
Engber, T. M., Susel, Z., Juncos, J. L., and Chase, T. N. (1989). Continuous and intermittent levodopa differentially affect rotation induced by D1 and D2 dopamine agonists. Eur. J. Pharmacol. 168, 291–298. doi: 10.1016/0014-2999(89)90790-5
Fuchs, T., Saunders-Pullman, R., Masuho, I., Luciano, M. S., Raymond, D., Factor, S., et al. (2013). Mutations in GNAL cause primary torsion dystonia. Nat. Genet. 45, 88–92. doi: 10.1038/ng.2496
Fuxe, K., Marcellino, D., Genedani, S., and Agnati, L. (2007). Adenosine A2A receptors, dopamine D2 receptors and their interactions in Parkinson’s disease. Mov. Disord. 22, 1990–2017. doi: 10.1002/mds.21440
Gerfen, C. R., and Surmeier, D. J. (2011). Modulation of striatal projection systems by dopamine. Annu. Rev. Neurosci. 34, 441–466. doi: 10.1146/annurev-neuro-061010-113641
Gerfen, C. R., Miyachi, S., Paletzki, R., and Brown, P. (2002). D1 dopamine receptor supersensitivity in the dopamine-depleted striatum results from a switch in the regulation of ERK1/2/MAP kinase. J. Neurosci. 22, 5042–5054.
Girault, J. A., Raisman-Vozari, R., Agid, Y., and Greengard, P. (1989). Striatal phosphoproteins in Parkinson disease and progressive supranuclear palsy. Proc. Natl. Acad. Sci. U S A 86, 2493–2497. doi: 10.1073/pnas.86.7.2493
Goto, S., Hirano, A., and Matsumoto, S. (1989). Subdivisional involvement of nigrostriatal loop in idiopathic Parkinson’s disease and striatonigral degeneration. Ann. Neurol. 26, 766–770. doi: 10.1002/ana.410260613
Goto, S., Kawarai, T., Morigaki, R., Okita, S., Koizumi, H., Nagahiro, S., et al. (2013). Defects in the striatal neuropeptide Y system in X-linked dystonia-parkinsonism. Brain 136, 1555–1567. doi: 10.1093/brain/awt084
Graybiel, A. M. (2008). Habits, rituals, and the evaluative brain. Annu. Rev. Neurosci. 31, 359–387. doi: 10.1146/annurev.neuro.29.051605.112851
Guridi, J., González-Redondo, R., and Obeso, J. A. (2012). Clinical features, pathophysiology, and treatment of levodopa-induced dyskinesias in Parkinson’s disease. Parkinsons Dis. 2012:943159. doi: 10.1155/2012/943159
Guttman, M., and Seeman, P. (1985). L-dopa reverses the elevated density of D2 dopamine receptors in Parkinson’s disease striatum. J. Neural Transm. 64, 93–103. doi: 10.1007/bf01245971
Harvey, J., and Lacey, M. G. (1997). A postsynaptic interaction between dopamine D1 and NMDA receptors promotes presynaptic inhibition in the rat nucleus accumbens via adenosine release. J. Neurosci. 17, 5271–5280.
Herrera-Marschitz, M., Luthman, J., and Ferré, S. (1994). Unilateral neonatal intracerebroventricular 6-hydroxydopamine administration in rats: II. Effects on extracellular monoamine, acetylcholine and adenosine levels monitored with in vivo microdialysis. Psychopharmacology 116, 451–456. doi: 10.1007/bf02247477
Hervé, D. (2011). Identification of a specific assembly of the G protein Golf as a critical and regulated module of dopamine and adenosine-activated cAMP pathways in the striatum. Front. Neuroanat. 5:48. doi: 10.3389/fnana.2011.00048
Hervé, D., Le Moine, C., Corvol, J. C., Belluscio, L., Ledent, C., Fienberg, A. A., et al. (2001). Gα(olf) levels are regulated by receptor usage and control dopamine and adenosine action in the striatum. J. Neurosci. 21, 4390–4399.
Hervé, D., Lévi-Strauss, M., Marey-Semper, I., Verney, C., Tassin, J. P., Glowinski, J., et al. (1993). Golf and Gs in rat basal ganglia: possible involvement of Golf in the coupling of dopamine D1 receptor with adenylyl cyclase. J. Neurosci. 13, 2237–2248.
Huot, P., Johnston, T. H., Koprich, J. B., Fox, S. H., and Brotchie, J. M. (2013). The pharmacology of L-DOPA-induced dyskinesia in Parkinson’s disease. Pharmacol. Rev. 65, 171–222. doi: 10.1124/pr.111.005678
Hurley, M. J., Mash, D. C., and Jenner, P. (2001). Dopamine D1 receptor expression in human basal ganglia and changes in Parkinson’s disease. Mol. Brain Res. 87, 271–279. doi: 10.1016/s0169-328x(01)00022-5
Jenner, P. (2003). A2A antagonists as novel non-dopaminergic therapy for motor dysfunction in PD. Neurology 61, S32–S38. doi: 10.1212/01.WNL.0000095209.59347.79
Jenner, P. (2008). Molecular mechanisms of L-DOPA-induced dyskinesia. Nat. Rev. Neurosci. 9, 665–677. doi: 10.1038/nrn2471
Jenner, P., Mori, A., Hauser, R., Morelli, M., Fredholm, B. B., and Chen, J. F. (2009). Adenosine, adenosine A2A antagonists and Parkinson’s disease. Parkinsonism Relat. Disord. 15, 406–413. doi: 10.1016/j.parkreldis.2008.12.006
Kanda, T., and Uchida, S. (2014). Clinical/pharmacological aspect of adenosine A2A receptor antagonist for dyskinesia. Int. Rev. Neurobiol. 119, 127–150. doi: 10.1016/B978-0-12-801022-8.00006-4
Kashihara, K., Manabe, Y., Shiro, Y., Warita, H., and Abe, K. (2000). Effects of repeated methyl levodopa administration on apomorphine sensitivity of rotational behavior and striatal Fos expression of rats with unilateral 6-OHDA lesions. Neurosci. Res. 38, 273–279. doi: 10.1016/s0168-0102(00)00167-x
Kebabian, J. W., and Calne, D. B. (1979). Multiple receptors for dopamine. Nature 277, 93–96. doi: 10.1038/277093a0
Kish, S. J., Shannak, K., and Hornykiewicz, O. (1988). Uneven pattern of dopamine loss in the striatum of patients with idiopathic Parkinson’s disease. N. Engl. J. Med. 318, 876–880. doi: 10.1056/nejm198804073181402
Kondo, T., Mizuno, Y., and Japanese Istradefylline Study Group. (2015). A long-term study of istradefylline safety and efficacy in patients with Parkinson disease. Clin. Neuropharmacol. 38, 41–46. doi: 10.1097/WNF.0000000000000073
Kreitzer, A. C. (2009). Physiology and pharmacology of striatal neurons. Annu. Rev. Neurosci. 32, 127–147. doi: 10.1146/annurev.neuro.051508.135422
Kull, B., Svenningsson, P., and Fredholm, B. B. (2000). Adenosine A2A receptors are colocalized with and activate Golf in rat striatum. Mol. Pharmacol. 58, 771–777. doi: 10.1124/mol.58.4.771
LaHoste, G. J., and Marshall, J. F. (1994). Rapid development of D1 and D2 dopamine receptor sensitivity are indicated by striatal and pallidal Fos expression. Neurosci. Lett. 179, 153–156. doi: 10.1016/0304-3940(94)90957-1
Marcotte, E. R., Sullivan, R. M., and Mishra, R. K. (1994). Striatal G-proteins: effects of unilateral 6-hydroxydopamine lesions. Neurosci. Lett. 169, 195–198. doi: 10.1016/0304-3940(94)90390-5
Marshall, J. F., Cole, B. N., and LaHoste, G. J. (1993). Dopamine D2 receptor control of pallidal fos expression: comparisons between intact and 6-hydroxydopamine-treated hemispheres. Brain Res. 632, 308–313. doi: 10.1016/0006-8993(93)91166-p
Missale, C., Nash, S. R., Robinson, S. W., Jaber, M., and Caron, M. G. (1998). Dopamine receptors: from structure to function. Physiol. Rev. 78, 189–225.
Morigaki, R., and Goto, S. (2015). Postsynaptic density protein 95 in the striosome and matrix compartments of the human neostriatum. Front. Neuroanat. 9:154. doi: 10.3389/fnana.2015.00154
Morigaki, R., and Goto, S. (2016). Putaminal mosaic visualized by tyrosine hydroxylase immunohistochemistry in the human neostriatum. Front. Neuroanat. 10:34. doi: 10.3389/fnana.2016.00034
Nash, J. E., and Brotchie, J. M. (2000). A common signaling pathway for striatal NMDA and adenosine A2a receptors: implications for the treatment of Parkinson’s disease. J. Neurosci. 20, 7782–7789.
Nishino, N., Kitamura, N., Hashimoto, T., and Tanaka, C. (1993). Transmembrane signaling systems in the brain of patients with Parkinson’s disease. Rev. Neurosci. 4, 213–222. doi: 10.1515/REVNEURO.1993.4.2.213
Nomoto, M., Kaseda, S., Iwata, S., Shimizu, T., Fukuda, T., and Nakagawa, S. (2000). The metabolic rate and vulnerability of dopaminergic neurons and adenosine dynamics in the cerebral cortex, nucleus accumbens, caudate nucleus, and putamen of the common marmoset. J. Neurol. 247, V16–V22. doi: 10.1007/pl00007779
Okita, S., Morigaki, R., Koizumi, H., Kaji, R., Nagahiro, S., and Goto, S. (2012). Cell type-specific localization of optineurin in the striatal neurons of mice: implications for neuronal vulnerability in Huntington’s disease. Neuroscience 202, 363–370. doi: 10.1016/j.neuroscience.2011.11.059
Pavón, N., Martín, A. B., Mendialdua, A., and Moratalla, R. (2006). ERK phosphorylation and fosB expression are associated with L-dopa-induced dyskinesia in hemiparkinsonian mice. Biol. Psychiatry 59, 64–74. doi: 10.1016/j.biopsych.2005.05.044
Paxinos, G., and Franklin, K. B. J. (2001). The Mouse Brain in Stereotaxic Coordinates. 2nd Edn. San Diego, CA: Academic Press.
Penit-Soria, J., Durand, C., Besson, M. J., and Hervé, D. (1997). Levels of stimulatory G protein are increased in the rat striatum after neonatal lesion of dopamine neurons. Neuroreport 8, 829–833. doi: 10.1097/00001756-199703030-00005
Pinna, A., Corsi, C., Carta, A. R., Valentini, V., Pedata, F., and Morelli, M. (2002). Modification of adenosine extracellular levels and adenosine A2A receptor mRNA by dopamine denervation. Eur. J. Pharmacol. 446, 75–82. doi: 10.1016/s0014-2999(02)01818-6
Ramlackhansingh, A. F., Bose, S. K., Ahmed, I., Turkheimer, F. E., Pavese, N., and Brooks, D. J. (2011). Adenosine 2A receptor availability in dyskinetic and nondyskinetic patients with Parkinson’s disease. Neurology 76, 1811–1816. doi: 10.1212/WNL.0b013e31821ccce4
Rangel-Barajas, C., Silva, I., Lopéz-Santiago, L. M., Aceves, J., Erlij, D., and Florán, B. (2011). L-DOPA-induced dyskinesia in hemiparkinsonian rats is associated with up-regulation of adenylyl cyclase type V/VI and increased GABA release in the substantia nigra reticulata. Neurobiol. Dis. 41, 51–61. doi: 10.1016/j.nbd.2010.08.018
Rascol, O., Brooks, D. J., Korczyn, A. D., De Deyn, P. P., Clarke, C. E., Lang, A. E., et al. (2006). Development of dyskinesias in a 5-year trial of ropinirole and L-dopa. Mov. Disord. 21, 1844–1850. doi: 10.1002/mds.20988
Rinne, U. K., Lönnberg, P., and Koskinen, V. (1981). Dopamine receptors in the Parkinsonian brain. J. Neural Transm. 51, 97–106. doi: 10.1007/bf01664007
Ruiz-DeDiego, I., Naranjo, J. R., Hervé, D., and Moratalla, R. (2015). Dopaminergic regulation of olfactory type G-protein α subunit expression in the striatum. Mov. Disord. 30, 1039–1049. doi: 10.1002/mds.26197
Rylander, D., Recchia, A., Mela, F., Dekundy, A., Danysz, W., and Cenci, M. A. (2009). Pharmacological modulation of glutamate transmission in a rat model of L-DOPA-induced dyskinesia: effects on motor behavior and striatal nuclear signaling. J. Pharmacol. Exp. Ther. 330, 227–235. doi: 10.1124/jpet.108.150425
Sakagami, H., Sawamura, Y., and Kondo, H. (1995). Synchronous patchy pattern of gene expression for adenylyl cyclase and phosphodiesterase but discrete expression for G-protein in developing rat striatum. Mol. Brain Res. 33, 185–191. doi: 10.1016/0169-328x(95)00123-a
Sako, W., Morigaki, R., Nagahiro, S., Kaji, R., and Goto, S. (2010). Olfactory type G-protein α subunit in striosome-matrix dopamine systems in adult mice. Neuroscience 170, 497–502. doi: 10.1016/j.neuroscience.2010.06.072
Santini, E., Alcacer, C., Cacciatore, S., Heiman, M., Hervé, D., Greengard, P., et al. (2009). L-DOPA activates ERK signaling and phosphorylates histone H3 in the striatonigral medium spiny neurons of hemiparkinsonian mice. J. Neurochem. 108, 621–633. doi: 10.1111/j.1471-4159.2008.05831.x
Santini, E., Valjent, E., Usiella, A., Carta, M., Borgkvist, A., Girault, J. A., et al. (2007). Critical involvement of cAMP/DARPP-32 and extracellular signal-regulated protein kinase signaling in L-DOPA-induced dyskinesia. J. Neurosci. 27, 6995–7005. doi: 10.1523/JNEUROSCI.0852-07.2007
Sato, K., Sumi-Ichinose, C., Kaji, R., Ikemoto, K., Nomura, T., Nagatsu, I., et al. (2008). Differential involvement of striosome and matrix dopamine systems in a transgenic model of dopa-responsive dystonia. Proc. Natl. Acad. Sci. U S A 105, 12551–12556. doi: 10.1073/pnas.0806065105
Schiffmann, S. N., Jacobs, O., and Vanderhaeghen, J. J. (1991). Striatal restricted adenosine A2 receptor (RDC8) is expressed by enkephalin but not by substance P neurons: an in situ hybridization histochemistry study. J. Neurochem. 57, 1062–1067. doi: 10.1111/j.1471-4159.1991.tb08257.x
Schwarzschild, M. A., Agnati, L., Fuxe, K., Chen, J. F., and Morelli, M. (2006). Targeting adenosine A2A receptors in Parkinson’s disease. Trends Neurosci. 29, 647–654. doi: 10.1016/j.tins.2006.09.004
Shinotoh, H., Inoue, O., Hirayama, K., Aotsuka, A., Asahina, M., Suhara, T., et al. (1993). Dopamine D1 receptors in Parkinson’s disease and striatonigral degeneration: a positron emission tomography study. J. Neurol. Neurosurg. Psychiatry 56, 467–472. doi: 10.1136/jnnp.56.5.467
Söderberg, O., Gullberg, M., Jarvius, M., Ridderstråle, K., Leuchowius, K. J., Jarvius, J., et al. (2006). Direct observation of individual endogenous protein complexes in situ by proximity ligation. Nat. Methods 3, 995–1000. doi: 10.1038/nmeth947
Svenningsson, P., Le Moine, C., Fisone, G., and Fredholm, B. B. (1999). Distribution, biochemistry and function of striatal adenosine A2A receptors. Prog. Neurobiol. 59, 355–396. doi: 10.1016/s0301-0082(99)00011-8
Tanabe, A., Yamamura, Y., Kasahara, J., Morigaki, R., Kaji, R., and Goto, S. (2014). A novel tyrosine kinase inhibitor AMN107 (nilotinib) normalizes striatal motor behaviors in a mouse model of Parkinson’s disease. Front. Cell. Neurosci. 8:50. doi: 10.3389/fncel.2014.00050
Thobois, S., Vingerhoets, F., Fraix, V., Xie-Brustolin, J., Mollion, H., Costes, N., et al. (2004). Role of dopaminergic treatment in dopamine receptor down-regulation in advanced Parkinson disease: a positron emission tomographic study. Arch. Neurol. 61, 1705–1709. doi: 10.1001/archneur.61.11.1705
Turjanski, N., Lees, A. J., and Brooks, D. J. (1997). in vitro studies on striatal dopamine D1 and D2 site binding in L-dopa-treated Parkinson’s disease patients with and without dyskinesias. Neurology 49, 717–723. doi: 10.1212/WNL.49.3.717
Keywords: olfactory type G-protein α subunit, dopamine, striatum, Parkinson’s disease, L-DOPA-induced dyskinesia
Citation: Morigaki R, Okita S and Goto S (2017) Dopamine-Induced Changes in Gαolf Protein Levels in Striatonigral and Striatopallidal Medium Spiny Neurons Underlie the Genesis of L-DOPA-Induced Dyskinesia in Parkinsonian Mice. Front. Cell. Neurosci. 11:26. doi: 10.3389/fncel.2017.00026
Received: 01 December 2016; Accepted: 26 January 2017;
Published: 10 February 2017.
Edited by:
Hansen Wang, University of Toronto, CanadaReviewed by:
Emmanuel Valjent, Centre National de la Recherche Scientifique (CNRS), FranceDenis Hervé, Institut National de la Santé et de la Recherche Médicale (INSERM), France
Véronique Sgambato-Faure, Centre National de la Recherche Scientifique (CNRS), France
Copyright © 2017 Morigaki, Okita and Goto. This is an open-access article distributed under the terms of the Creative Commons Attribution License (CC BY). The use, distribution and reproduction in other forums is permitted, provided the original author(s) or licensor are credited and that the original publication in this journal is cited, in accordance with accepted academic practice. No use, distribution or reproduction is permitted which does not comply with these terms.
*Correspondence: Satoshi Goto, sgoto@tokushima-u.ac.jp