The corticostriatal and corticosubthalamic pathways: two entries, one target. So what?
- 1 Yerkes National Primate Research Center, Emory University, Atlanta, GA, USA
- 2 Department of Neurology, Emory University, Atlanta, GA, USA
The basal ganglia receive cortical inputs through two main stations – the striatum and the subthalamic nucleus (STN). The information flowing along the corticostriatal system is transmitted to the basal ganglia circuitry via the “direct and indirect” striatofugal pathways, while information that flows through the STN is transmitted along the so-called “hyperdirect” pathway. The functional significance of this dual entry system is not clear. Although the corticostriatal system has been thoroughly characterized anatomically and electrophysiologically, such is not the case for the corticosubthalamic system. In order to provide further insights into the intricacy of this complex anatomical organization, this review examines and compares the anatomical and functional organization of the corticostriatal and corticosubthalamic systems, and highlights some key issues that must be addressed to better understand the mechanisms by which these two neural systems may interact to regulate basal ganglia functions and dysfunctions.
Introduction
The direct and indirect pathway model of the basal ganglia has traditionally been the most authenticated working basal ganglia model. According to this model, cortical inputs enter the striatum and proceed to the output nuclei of the basal ganglia [internal globus pallidus (GPi) and the substantia nigra pars reticulata (SNr)] via two distinct pathways, enroute to the thalamus which projects back to the cerebral cortex. The “direct pathway” refers to the monosynaptic connection from the striatum to GPi/SNr; whereas the “indirect pathway” is the polysynaptic pathway where the order of connectivity is striatum – external globus pallidus (GPe) – subthalamic nucleus (STN) – GPi/SNr (Albin et al., 1989; Delong, 1990). Normal basal ganglia functions are achieved when there is a balance between the activities of these two pathways. Movement disorders of basal ganglia origin, both hypokinetic (e.g., Parkinson’s disease) and hyperkinetic (e.g., Huntington’s disease), are thought to result from imbalanced activities between the two pathways; with the polarity of the imbalance determining the kinetics of the disorder (Albin et al., 1989).
In addition to the corticostriatal inputs, the basal ganglia also receive direct cortical information at the level of the STN, which further gets relayed to the GPi/SNr, constituting the “hyperdirect pathway” (Monakow et al., 1978; Nambu et al., 1996; Figures 1 and 2). Although the existence of this system has long been recognized, its detailed anatomical organization, integration within the functional circuitry of the basal ganglia and significance in the pathophysiology of the basal ganglia network in movement disorders remains highly hypothetical and poorly understood.
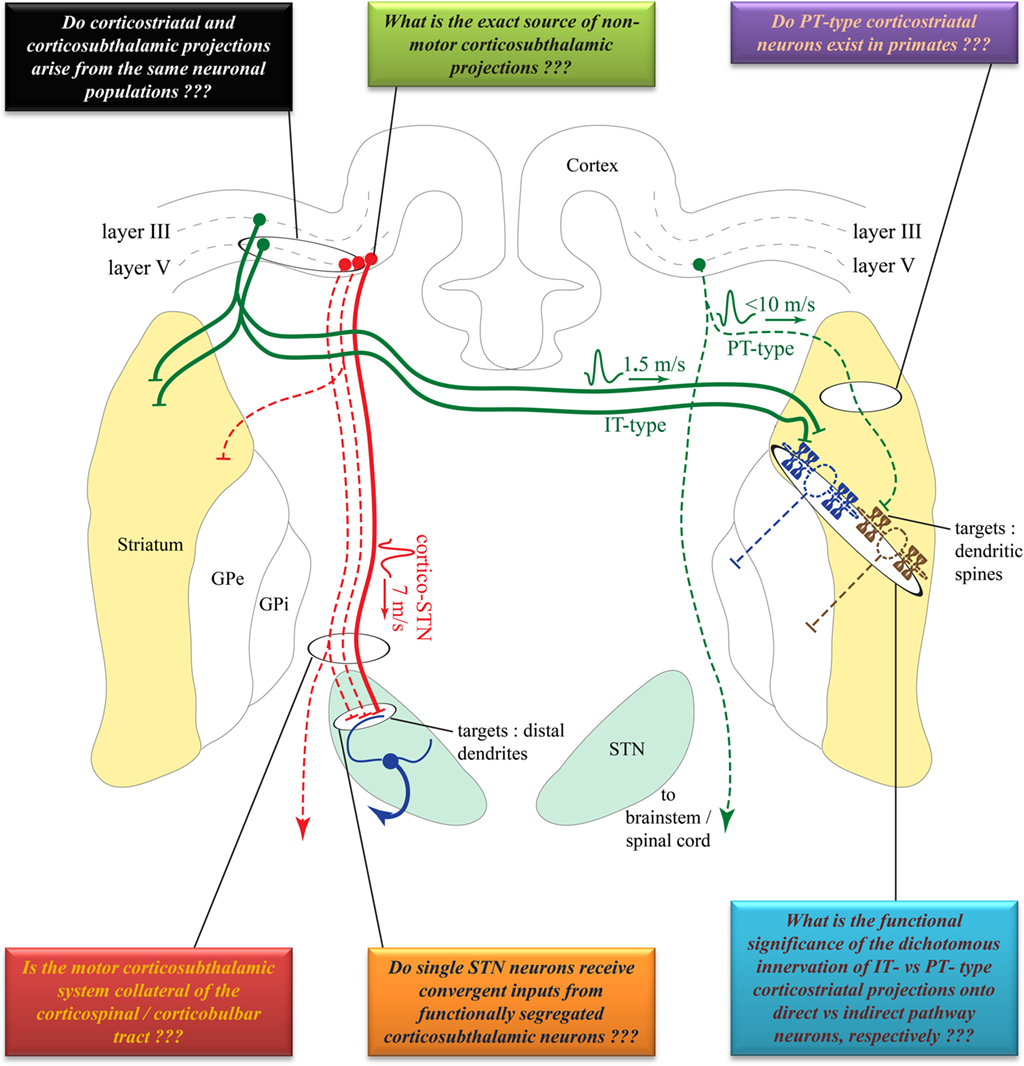
Figure 1. Comparison of the cortical sources, conduction velocities and synaptic targets of corticostriatal versus corticosubthalamic projections. Solid lines indicate well established projections. Dotted lines indicate projections that need to be confirmed in future studies. Colored boxes summarize some of the unanswered questions pertaining to the anatomical, synaptic, and functional organization of these two pathways. See text for abbreviations. Anatomical structures are not to scale, but depicted solely for illustrative purposes and are not necessarily in the same coronal plane. Some connections have been purposefully omitted to decrease complexity.
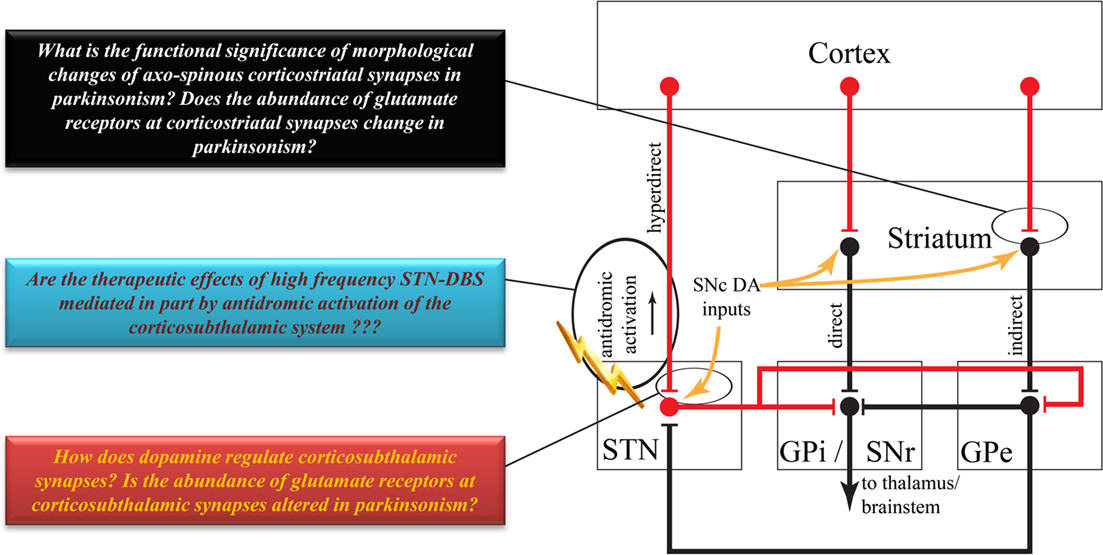
Figure 2. Highly simplified overview of the classical model of the basal ganglia circuitry. Red lines indicate excitatory projections. Black lines indicate inhibitory projections. Some brainstem connections have been omitted for the sake of simplicity. Colored boxes highlight some key issues to be addressed about the anatomy and function of the corticostriatal and corticosubthalamic systems. See text for abbreviations.
In this review, we compare the anatomical and functional organization of the two main cortical entry systems to the basal ganglia, and critically examine their respective roles in the integration and processing of motor and non-motor information that flows through the cortico-basal ganglia–thalamocortical loops in normal and pathological conditions. At the end of each section, we highlight some relevant anatomical and functional issues that should be addressed to increase our current knowledge of the complementary roles corticostriatal and corticosubthalamic entry systems to the basal ganglia play in normal and diseased states.
Neuronal Sources and Conduction Velocities of Corticostriatal Versus Corticosubthalamic Projections
Sources of the Corticostriatal System
Corticostriatal projections typically arise from small to medium sized layer III and V pyramidal neurons in functionally diverse cortical areas in monkeys, dogs, cats, and rodents (Kemp and Powell, 1970; Kitai et al., 1976; Jones et al., 1977; Oka, 1980; Veening et al., 1980; Royce, 1982; Goldman-Rakic and Selemon, 1986; Tanaka, 1987; Mcgeorge and Faull, 1989). In rodents, corticostriatal neurons are categorized into two main types: the intratelencephalic (IT) and the pyramidal tract (PT) neurons (Jinnai and Matsuda, 1979; Landry et al., 1984; Wilson, 1987; Cowan and Wilson, 1994; Levesque et al., 1996a,b; Levesque and Parent, 1998; Wright et al., 1999, 2001; Zheng and Wilson, 2002; Reiner et al., 2003; Parent and Parent, 2006). The IT neurons, of which axonal projections are confined to the ipsilateral and contralateral cortex and striatum, are mainly located in layer III and upper layer V of the rat cortex. In contrast, PT neurons are located in the lower layer V, and send long descending axonal projections to the brainstem and spinal cord from which originate thin axon collaterals that innervate the striatum (Reiner et al., 2003; Figure 1). A unidirectional pattern of physiological connectivity from IT to PT corticostriatal neurons has been demonstrated in cortical slices (Morishima and Kawaguchi, 2006).
Although single-axon tracing studies have identified distinct populations of corticostriatal projection neurons reminiscent of the rodent IT and PT neurons in monkeys (Parent and Parent, 2006), this dual corticostriatal system is not supported by electrophysiological studies in non-human primates. For instance, stimulation of the motor putamen and the PT does not or very rarely elicit antidromic activation of single neurons in the primary motor cortex (MI) and pre-motor cortices in monkeys (Bauswein et al., 1989; Turner and Delong, 2000), suggesting that the PT does not give off significant collaterals to the striatum (Figure 1). Even in rodents, the functional significance of the dichotomous IT and PT corticostriatal neurons system is not clearly understood. Anatomical evidence suggests that IT-type neurons preferentially innervate direct pathway striato-nigral neurons, whereas PT-type neurons mainly target indirect pathway striato-pallidal neurons (Lei et al., 2004). However, a recent in vivo electrophysiology study could not detect a significant PT-type corticostriatal input onto indirect striatofugal neurons. This study rather showed that IT-type corticostriatal neurons are the main excitatory drive to both direct and indirect striatofugal neurons (Ballion et al., 2008; Figure 1).
Sources of the Corticosubthalamic System
The corticosubthalamic projections originate primarily from cortical layer V neurons in rats and monkeys (Rouzaire-Dubois and Scarnati, 1985; Canteras et al., 1990; Nambu et al., 1996). Although overwhelming evidence points to an ipsilateral corticosubthalamic system (Afsharpour, 1985; Feger et al., 1994), one electrophysiological study performed in unilaterally decorticated rats suggests that part of the corticosubthalamic tract may project contralaterally (Rouzaire-Dubois and Scarnati, 1985). However, a subsequent electrophysiological study in rats showed that contralateral cortical stimulation elicits long latency excitatory responses in the STN which, most likely, rely on oligosynaptic circuits rather than the fast monosynaptic corticosubthalamic connections that mediate ipsilateral effects (Fujimoto and Kita, 1993). Preliminary data suggest that axon collaterals of the corticospinal tract innervate the STN in cats (Iwahori, 1978; Giuffrida et al., 1985). However, this issue must be thoroughly investigated in rats and monkeys using sensitive anatomical and electrophysiological methods. Although part of the corticosubthalamic tract projecting to the motor territory of the STN could, indeed, be axon collaterals of the descending corticospinal and corticobulbar tracts, it is not clear if non-motor projections to the STN originate from axon collaterals of cortical efferents to other cortical or subcortical targets, or from a specific subset of corticosubthalamic neurons (Figure 1). In light of recent data suggesting that some of the therapeutic benefits, or side effects, of STN deep brain stimulation could be attributed to antidromic activation of motor (Li et al., 2007) versus non-motor corticosubthalamic systems (Drouot et al., 2004; Temel et al., 2006; Li et al., 2007; Gradinaru et al., 2009), respectively, a detailed knowledge of the exact sources and degree of collateralization of corticosubthalamic axons is essential to a deeper understanding of the mechanisms and anatomical substrates through which STN DBS mediates its wanted and unwanted effects (Figure 2).
Do the Corticostriatal and Corticosubthalamic Systems Originate from Single Cortical Neurons?
In monkeys, striatal stimulation results in monosynaptic short latency spike discharge (10–15 m/s) in the STN (Ohye et al., 1976). Considering that there is no clear evidence for the existence of a striatosubthalamic projection system; some of these short latency responses could possibly be mediated by axons collaterals of single cortical neurons innervating both the striatum and STN. Such short latency responses could also be attributed to activation of fibers of passage leading to the STN. Nevertheless, the extent of collateralization of single cortical projections to the striatum and the STN is unclear, and must be directly assessed using reliable anatomical approaches (Figure 1). In that regard, a recent single-axon tracing study in non-human primates has revealed the existence of corticosubthalamic projections that descend through the cerebral peduncle or the red nucleus, but did not describe any cortical neurons innervating both the striatum and STN (Parent and Parent, 2006). However, a double retrograde labeling study in rats suggested that a subset of individual cortical neurons project to both structures (Feger et al., 1994).
Further evidence for a distinct origin of corticostriatal and corticosubthalamic projection systems is suggested by the different conduction velocities of these axonal projections. In rats, the mean conduction velocity of hyperdirect corticosubthalamic axons (∼7 m/s) is much faster than that of the IT-type corticostriatal axons (∼1.5 m/s), but is slightly slower than the PT-type corticostriatal axons (<10 m/s; Cowan and Wilson, 1994; Mahon et al., 2001; Slaght et al., 2004; Paz et al., 2005). However, the conduction velocity value of the PT-type corticostriatal axons must be interpreted cautiously because of diameter differences between the rather thin corticostriatal axon collaterals that detach from the large-sized PT main descending axon to the brainstem. Another important aspect to consider is the fact that the speed of conduction of the corticospinal tract (∼11.4 m/s in rats, with the fastest reaching ∼19 m/s; Mediratta and Nicoll, 1983) is much faster than either corticosubthalamic or corticostriatal axons, thereby suggesting that these projections most likely originate from distinct neuronal populations, though this remains to be demonstrated (Figure 1). The functional relevance of these different rates of conduction toward the transmission and integration of information flow through the corticostriatal and corticosubthalamic systems is discussed below.
To better understand the origins and potential sites of cross-talk between corticostriatal and corticosubthalamic neurons, the following critical issues must be addressed (Figure 1):
(1) Determine the exact sources and innervation patterns of corticosubthalamic projections to motor and non-motor regions of the STN.
(2) Determine the proportion of motor corticosubthalamic axons that are collaterals of the corticospinal and corticobulbar tracts in primates.
(3) Determine the degree of collateralization of single corticofugal axons to the striatum and the STN.
(4) Determine the extent of cross-talk between corticostriatal and corticosubthalamic neurons at the cortical level.
Synaptic Microcircuitry of Corticostriatal Versus Corticosubthalamic Systems
Cortical inputs to the striatum are relatively dense and preferentially target medium spiny neurons (MSNs). In rats, each MSN receives approximately 5000 cortical inputs (Kincaid et al., 1998), that form asymmetric synapses almost exclusively on spine heads (∼90%; Kemp and Powell, 1971; Xu et al., 1989; Raju et al., 2006, 2008). It is clear that the corticostriatal system represents, by far, the most massive source of synaptic inputs to striatal projection neurons (Ingham et al., 1998; Raju et al., 2008). In contrast, the corticosubthalamic projection is much less profuse, and gives rise to a rather sparse population of terminals that form asymmetric synapses only with the distal dendrites of STN neurons (Bevan et al., 1995; Mathai et al., 2010). The main source of synaptic inputs to STN neurons is from the GPe, which provides a massive GABAergic innervation that spreads across the whole somatodendritic domain of single STN neurons (Smith et al., 1990, 1998). Despite its relative scarcity and distal location, corticosubthalamic afferents are considered as a major driving force of STN neurons, and a key source of inputs through which motor cortical information reaches basal ganglia output neurons (Nambu et al., 2002; Magill et al., 2004). Complex synaptic mechanisms and membrane properties of STN neurons have been suggested to explain how sparse, distally located cortical inputs could mediate powerful excitatory effects upon STN neurons despite the massive and tonically active inhibitory influences from the GPe (Bevan et al., 2002, 2007).
In addition to the prevalence and distribution of cortical inputs, the abundance, subsynaptic localization, pharmacological properties, and plasticity of postsynaptic glutamate receptors associated with these afferents are other factors that could contribute to the strength of corticostriatal and corticosubthalamic inputs (Figure 2). Striatal projection neurons express a wide variety of AMPA and NMDA receptor subunits and multiple subtypes of metabotropic glutamate receptors (mGluRs). At the electron microscopic level, these receptor proteins are profusely expressed along the plasma membrane of dendritic spines and dendritic shafts where most cortical inputs are located, though most mGluRs and a significant contingent of AMPA and NMDA receptor subunits are also heavily localized extrasynaptically (Paquet and Smith, 2003; Fujiyama et al., 2004; Galvan et al., 2006). Although the functional role of these receptors in corticostriatal transmission and long term plasticity has been demonstrated (Calabresi et al., 2007; Wickens, 2009), the relative abundance of specific receptor subunits at individual cortical synapses remains unknown.
Subthalamic nucleus neurons are also enriched with ionotropic NMDA and AMPA receptor subunits as well as with group I mGluRs (i.e., mGluR1 and mGluR5), located along the plasma membrane of STN neurons, some of those being in the main body or peri-synaptic to asymmetric glutamatergic synapses (Clarke and Bolam, 1998; Kuwajima et al., 2004). However, there is no detailed study of the expression level of any glutamate receptor subtypes at corticosubthalamic synapses compared with other glutamatergic efferents to the STN (i.e., vesicular glutamate transporter 2 – positive terminals from the thalamus and pedunculopontine nucleus). Thus, to better understand the synaptic mechanisms by which the microcircuitry and relative expression of specific receptor subtypes contribute to the functional effects of corticostriatal versus corticosubthalamic afferents upon their target neurons, a detailed quantitative assessment of the morphological and neurochemical features of these synapses must be achieved (Figure 2). Some of the key information to be gathered include:
(1) A detailed quantitative analysis of the prevalence and distribution of cortical inputs onto the dendrites of single STN neurons.
(2) A detailed comparison of the relative abundance of specific glutamate receptor subtypes or subunits at individual corticostriatal versus corticosubthalamic synapses (Figure 3).
(3) Electrophysiological assessment of the strength, properties and long term plasticity of corticosubthalamic synapses.
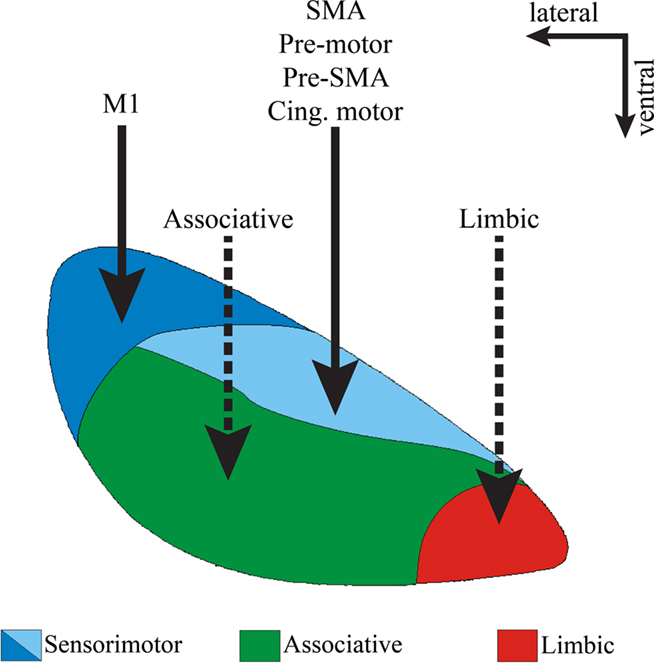
Figure 3. Functional domains of the STN. Coronal view of the primate STN to illustrate the topographical organization of cortical afferents to various functional regions of the nucleus defined by their reciprocal connections with the globus pallidus and motor cortical areas. Solid lines indicate well established corticosubthalamic projections from motor and pre-motor cortices. Dotted lines indicate putative projections that need further confirmation. Abbreviations: cing, cingulate.
Functional Segregation of Corticostriatal Versus Corticosubthalamic Pathways
Corticostriatal Inputs are Functionally Segregated
Multiple cortical areas project to the striatum in a highly topographic manner creating functionally segregated maps (Alexander et al., 1986). The post-commissural dorsolateral putamen primarily receives sensorimotor cortical afferents, most of the pre-commissural putamen and caudate nucleus receive afferents from associative cortical regions, whereas the limbic and paralimbic cortices, the amygdala and the hippocampus innervate the nucleus accumbens, the ventral caudate, and ventral putamen (Parent and Hazrati, 1995). Although the functional territories in the striatum are largely segregated, somatosensory and motor cortical information representing the same body parts converge onto overlapping regions in the putamen (Flaherty and Graybiel, 1991, 1993). There is no evidence for a similar overlap of somatosensory and motor cortical information in the STN. In fact, there are conflicting data regarding the existence of somatosensory, visual or auditory inputs to the STN from primary sensory cortices (Afsharpour, 1985; Canteras et al., 1988; Kolomiets et al., 2001), but it is worth noting that some sensory modalities can still reach the STN via other routes, such as the tectosubthalamic tract recently described in rodents (Coizet et al., 2009). Complex patterns of overlapping and interdigitation of corticostriatal projections from interconnected associative cortical areas have also been described in the caudate nucleus and ventral striatum of non-human primates (Yeterian and Van Hoesen, 1978; Selemon and Goldman-Rakic, 1985; Parthasarathy et al., 1992), but very little is known about the relative overlap or segregation of associative cortical inputs to the STN.
Is the Corticosubthalamic System Functionally Segregated?
Despite the limited knowledge about the organization of non-motor corticosubthalamic projections compared with the detailed analyzes of the corticostriatal systems, the STN is also topographically divided into functional territories, mainly based on its connections with specific functionally segregated regions of the globus pallidus (Figure 3). The dorsolateral and dorsomedial STN primarily processes motor-related information, the ventrolateral STN is the main target of associative-related pallidal inputs, while the ventromedial tip of the STN is primarily connected with limbic pallidal regions including the ventral pallidum (Parent, 1990; Shink et al., 1996; Karachi et al., 2005; Smith, 2011). In primates, cortical inputs from the primary MI innervate the dorsolateral STN, while the supplementary motor area (SMA), pre-motor cortex (PM), and cingulate motor cortex (CM) send projections to the dorsomedial STN (Nambu et al., 1996, 1997; Takada et al., 2001; Figure 3).
However, there is only scarce anatomical evidence of direct cortical projections from associative and limbic cortices to the monkey STN (Monakow et al., 1978). In rodents, lesion studies (Eagle et al., 2008) have suggested that functional connections between the medial prefrontal cortex and the STN are necessary to perform tasks that involve cognitive and reward processing (Dias et al., 1996; Chudasama et al., 2003; Baunez and Gubellini, 2010; Eagle and Baunez, 2010). As of date, the topography and extent of innervation of non-motor cortical inputs to the primate STN is not known (Figure 1), but recent diffusion-weighed magnetic resonance imaging studies suggest connections between high order associative cortical regions and the STN in humans (Aron et al., 2007). In addition, data from our laboratory indicate that the relative density of cortical terminals in non-motor regions of the monkey STN is as high as that in the motor STN, thereby suggesting significant associative and limbic cortical inputs to the non-human primate STN (Mathai et al., 2010).
Do Single STN Neurons Integrate Functionally Segregated Information Flowing Along the Corticosubthalamic System?
Despite the apparent anatomical segregation of motor, associative and limbic cortical inputs to the STN, the small size of the nucleus combined with the large extent of the dendritic tree of single STN neurons, open up the possibility for synaptic convergence of different cortical inputs onto single STN neurons, most particularly those located at the junction between different functional territories (Takada et al., 2001). STN neurons, indeed, harbor long dendrites, which, in primates, can extend as far as 600 μm from their parent cell bodies (Yelnik and Percheron, 1979; Figure 1). It is noteworthy that the extent of the dendritic domain of an individual STN neuron can cover about half, one-fifth, and one-ninth of the STN in the cat, monkey, and human, respectively (Yelnik and Percheron, 1979), thereby indicating that the functional segregation of the STN may increase in an ascending fashion as we compare cats, monkeys, and humans. In rats, some of the corticosubthalamic projections from functionally distinct cortical regions converge onto single STN neurons (Kolomiets et al., 2001), suggesting that the corticosubthalamic system is, indeed, more functionally convergent than the functionally segregated corticostriatal system, at least in rodents (Kolomiets et al., 2001). In line with this concept, it is worth noting that inputs from functionally segregated regions of the GP converge upon single STN neurons in rats (Bevan et al., 1997; Figure 1). In contrast, albeit extensive, the dendritic tree of striatal MSNs is much more restricted (200–260 μm in diameter in primates) and confined to the close vicinity of parent cell bodies (Graveland et al., 1985). Whether these anatomical differences in the dendritic arborization of striatal MSNs versus subthalamic neurons account for a higher level of convergence of functionally segregated cortical influences upon single STN than striatal neurons remains to be conclusively established, especially in primates (Figure 1).
Another anatomical feature that governs the extent of convergence or divergence of inputs upon their synaptic targets is their respective pattern of axonal arborization inside the target structure. Using single cell filling studies, these features have been studied for the corticostriatal system, but much remains to be done for the corticosubthalamic connections. In the monkey striatum, cortical axons split into about two to five branches upon entering the structure, and later arborize scarcely, but widely (Parent and Parent, 2006), thereby suggesting that each corticostriatal axon could target a large pool of striatal dendrites along its long tortuous course, but form a restricted number of synapses upon each of them. In rodents, PT-type neurons form focal clusters of fine processes and terminals, whereas the axonal projections of IT-type neurons arborize uniformly in the striatum (Wilson, 1987; Cowan and Wilson, 1994; Wright et al., 1999, 2001). As far as the corticosubthalamic pathway is concerned, very little is known about the extent and pattern of arborization of single cortical axons in the STN (Parent and Parent, 2006).
Thus, in order to assess and compare the degree of convergence between functionally segregated corticostriatal versus corticosubthalamic projections, the following issues must be addressed:
(1) Determine the sources and pattern of organization of direct sensory inputs to the monkey STN.
(2) Assess the degree of convergence of motor and somatosensory inputs related to the same body parts into the monkey STN.
(3) Analyze in detail the topographical organization of non-motor cortical inputs to the STN, especially in the monkey.
(4) Characterize the course and pattern of arborization of single motor and non-motor corticosubthalamic axons.
(5) Assess the anatomo-functional convergence of functionally distinct cortical inputs on single STN neurons.
Dopaminergic Regulation of Glutamatergic Corticostriatal Versus Corticosubthalamic Systems – Implications for Parkinson’s Disease Pathophysiology
The role of striatal dopamine has been explored in great detail and summarized in comprehensive reviews published over recent decades (Arbuthnott et al., 2000; Nicola et al., 2000; Reynolds and Wickens, 2002; Surmeier et al., 2007, 2009; Kreitzer and Malenka, 2008; Wickens, 2009). In the following account, we will only highlight a few points indicating the importance of dopamine in the regulation of corticostriatal activity so that it can be compared with our current knowledge of the potential effects of dopamine upon the corticosubthalamic system. In the classical models of the basal ganglia, dopamine regulates the balance between the activation of the direct and indirect striatofugal pathways (Wichmann and Delong, 1996). Striatal dopamine also plays a key role in mediating long term plasticity of glutamatergic corticostriatal synapses (Cragg, 2003; Picconi et al., 2003; Calabresi et al., 2007; Kreitzer and Malenka, 2008; Pawlak and Kerr, 2008). The loss of striatal dopamine in Parkinson’s disease leads to a major pruning of dendritic spines on striatal projection neurons (Ingham et al., 1989; Stephens et al., 2005; Zaja-Milatovic et al., 2005; Smith and Villalba, 2008; Villalba et al., 2009; Villalba and Smith, 2010), corresponding with a loss of corticostriatal terminals and a severe dysregulation and imbalance of activity between the direct and indirect striatofugal pathways (Delong, 1990; Pang et al., 2001; Liang et al., 2008), and a dramatic change in the long term plastic properties of corticostriatal synapses (Calabresi et al., 2007; Figures 1 and 2). There is also evidence that dopamine regulates the functional specificity of striatal projection neurons in response to cortical afferents, and that degeneration of the nigrostriatal dopaminergic system in PD underlies some of the pathophysiological patterns of activity striatal and other basal ganglia neurons display in parkinsonian condition (Calabresi et al., 1993, 2000; Florio et al., 1993; Onn and Grace, 1999; Onn et al., 2000; Strafella et al., 2005; Figure 2).
Albeit sparser than in the striatum, the STN also receives a dopaminergic innervation from collaterals of the nigrostriatal pathway, and STN neurons express various dopamine receptor subtypes (Lavoie et al., 1989; Hedreen, 1999; Augood et al., 2000; Francois et al., 2000; Smith and Kieval, 2000; Cragg et al., 2004; Smith and Villalba, 2008; Rommelfanger and Wichmann, 2010). Although there is evidence for physiological dopamine-mediated effects in the STN, functional interactions between the dopamine nigrosubthalamic system and corticosubthalamic afferents remain to be established (Figure 2). On the other hand, it is worth noting that the excitatory responses of SNr basal ganglia output neurons in response to cortical stimulation are augmented in 6-hydroxydopamine-treated parkinsonian rats compared to control animals (Belluscio et al., 2007). Whether this abnormal increased response of SNr neurons relies on changes in the dopamine-mediated regulation of the corticosubthalamic system in parkinsonism, remains to be established. Preliminary data from our laboratory have demonstrated a significant reduction in the density of vesicular glutamate transporter 1 – positive corticosubthalamic terminals in MPTP-treated parkinsonian monkeys (Mathai et al., 2010), thereby suggesting possible loss of cortical inputs to the STN in the parkinsonian state, as shown in the striatum (see above; Figure 2).
To further understand and compare the role played by dopamine in the regulation of the corticosubthalamic versus corticostriatal systems, the following points must be clarified (Figure 2):
(1) Does STN dopamine denervation induce changes in the number, microcircuitry and activity of corticosubthalamic inputs in parkinsonian condition?
(2) How do dopamine- and dopamine receptor-related drugs affect the strength and plastic properties of corticosubthalamic inputs?
(3) How do changes in dopaminergic innervation affect sensorimotor properties of STN neurons in response to cortical afferents in the parkinsonian state?
(4) Does STN dopamine denervation induce downstream regulatory changes in the expression, trafficking, and functional activity of dopamine and glutamate receptors that could influence corticosubthalamic transmission in parkinsonian condition?
Functional Interactions between the Corticostriatal and Corticosubthalamic Systems to Regulate the Selection of Basal Ganglia Motor Programs in Normal and Pathological Conditions
Ultimately, the significance of data discussed in this review relies on a better understanding of the functional interactions between information flowing along the corticostriatal and corticosubthalamic systems to mediate basal ganglia functions and dysfunctions in normal and diseased states. In that regard, a functional “center–surround model” of selection of motor programs in the basal ganglia has been proposed based on the temporal activation patterns of the hyperdirect corticosubthalamic pathway, and the direct corticostriatopallidal system (Mink, 1996; Nambu et al., 2000, 2002). According to this model, the cortical information flowing along the hyperdirect pathway is faster, and transmitted in a more diffuse manner than information flowing along the corticostriatal system to the GPi, thereby providing a general excitation over a large pool of basal ganglia output neurons not related to the selected motor act (i.e., the “surround neurons”). In contrast, a corollary signal transmitted along the direct corticostriatofugal pathway is much more focused and conveyed to a restricted pool of GPi neurons (i.e., the “center neurons”), that encode and transmit the information related to the desired motor acts to the thalamus and brainstem, thereby inducing focused inhibitory influences upon a pool of basal ganglia output neurons related to the motor act. Finally, a third corollary signal transmitted along the indirect corticostriatofugal pathway inhibits basal ganglia output neurons. Electrophysiological data have, indeed, demonstrated that cortical stimulation evokes a triphasic response in monkey GPi neurons (and rodent GP), including an early excitatory component induced by activation of the STN (Kita, 1992; Maurice et al., 1999; Nambu et al., 2000, 2002), prior to a slower inhibition generated by activation of the direct cortico-striato-GPi system followed by a late excitation most likely due to activation of the indirect cortico-striato-GPe–STN–GPi network (Kita, 1992; Maurice et al., 1999; Nambu et al., 2000, 2002). This attractive working hypothesis serves as an interesting foundation to further understand the possible interactions between the two cortical entry systems to the basal ganglia to control basal ganglia outflow.
However, as for any simplified models, part of it is speculative, and some of the assumptions made rely on anatomical and electrophysiological foundations that deserve some consideration. For instance, although some anatomical studies have proposed the existence of a diffuse subthalamopallidal system (Hazrati and Parent, 1992), as suggested in this model, others have demonstrated that the anatomical relationships between the STN and both pallidal segments are highly specific and topographic (Shink et al., 1996; Smith et al., 1998). In fact, recent single cell filling studies of individual subthalamopallidal and striato-pallidal neurons have revealed a high level of complexity of the axonal arborization of these two systems in the monkey GPi, demonstrating that projections from either systems can terminate in the GPi in a diffuse or focused manner (Parent et al., 1995; Sato et al., 2000). Another issue to be considered is the assumption that the subthalamopallidal system is active before movement onset in order to create the surround excitation proposed in this model. In monkeys, most STN neurons increase their firing around the time of movement onset or after the action during active step tracking movements (Wichmann et al., 1994; Delong and Wichmann, 2009), thereby reducing the likelihood that the corticosubthalamic projection is involved in the preparation of movements as suggested by the center–surround hypothesis. On the other hand, some human studies have shown that most STN neurons are active before self-paced movements in Parkinson’s disease (Paradiso et al., 2003).
To summarize, the functional mechanisms by which corticosubthalamic and corticostriatal projections interact to regulate motor, and possibly non-motor, behavior are complex, and necessitate further investigations.
Some of the key points that must be addressed to increase knowledge in this area:
(1) The localization and proportion of GPi neurons that display an early STN-mediated excitatory response in normal and parkinsonian state following stimulation of specific cortical areas.
(2) The changes in the temporal sequences of excitatory and inhibitory responses in GPi neurons following cortical activation in normal and parkinsonian state.
Conclusion
In this review, we highlighted some of the main anatomical, neurochemical, and functional features that characterize the two main routes of cortical entry to the basal ganglia circuitry, the corticostriatal, and the corticosubthalamic systems. Despite the large amount of information showing obvious differences in the functional anatomy of these two systems, our basic understanding of the temporal, spatial, and functional relationships through which these neural connections interact to mediate normal basal ganglia function, and their changes thereof in pathological conditions, are paramount in our quest of the mysterious functions of the basal ganglia in normal and diseased states.
Conflict of Interest Statement
The authors declare that the research was conducted in the absence of any commercial or financial relationships that could be construed as a potential conflict of interest.
References
Afsharpour, S. (1985). Topographical projections of the cerebral cortex to the subthalamic nucleus. J. Comp. Neurol. 236, 14–28.
Albin, R. L., Young, A. B., and Penney, J. B. (1989). The functional anatomy of basal ganglia disorders. Trends Neurosci. 12, 366–375.
Alexander, G. E., Delong, M. R., and Strick, P. L. (1986). Parallel organization of functionally segregated circuits linking basal ganglia and cortex. Annu. Rev. Neurosci. 9, 357–381.
Arbuthnott, G. W., Ingham, C. A., and Wickens, J. R. (2000). Dopamine and synaptic plasticity in the neostriatum. J. Anat. 196(Pt 4), 587–596.
Aron, A. R., Behrens, T. E., Smith, S., Frank, M. J., and Poldrack, R. A. (2007). Triangulating a cognitive control network using diffusion-weighted magnetic resonance imaging (MRI) and functional MRI. J. Neurosci. 27, 3743–3752.
Augood, S. J., Hollingsworth, Z. R., Standaert, D. G., Emson, P. C., and Penney, J. B. Jr. (2000). Localization of dopaminergic markers in the human subthalamic nucleus. J. Comp. Neurol. 421, 247–255.
Ballion, B., Mallet, N., Bezard, E., Lanciego, J. L., and Gonon, F. (2008). Intratelencephalic corticostriatal neurons equally excite striatonigral and striatopallidal neurons and their discharge activity is selectively reduced in experimental parkinsonism. Eur. J. Neurosci. 27, 2313–2321.
Baunez, C., and Gubellini, P. (2010). Effects of GPi and STN inactivation on physiological, motor, cognitive and motivational processes in animal models of Parkinson’s disease. Prog. Brain Res. 183, 235–258.
Bauswein, E., Fromm, C., and Preuss, A. (1989). Corticostriatal cells in comparison with pyramidal tract neurons: contrasting properties in the behaving monkey. Brain Res. 493, 198–203.
Belluscio, M. A., Riquelme, L. A., and Murer, M. G. (2007). Striatal dysfunction increases basal ganglia output during motor cortex activation in parkinsonian rats. Eur. J. Neurosci. 25, 2791–2804.
Bevan, M. D., Clarke, N. P., and Bolam, J. P. (1997). Synaptic integration of functionally diverse pallidal information in the entopeduncular nucleus and subthalamic nucleus in the rat. J. Neurosci. 17, 308–324.
Bevan, M. D., Francis, C. M., and Bolam, J. P. (1995). The glutamate-enriched cortical and thalamic input to neurons in the subthalamic nucleus of the rat: convergence with GABA-positive terminals. J. Comp. Neurol. 361, 491–511.
Bevan, M. D., Hallworth, N. E., and Baufreton, J. (2007). GABAergic control of the subthalamic nucleus. Prog. Brain Res. 160, 173–188.
Bevan, M. D., Magill, P. J., Terman, D., Bolam, J. P., and Wilson, C. J. (2002). Move to the rhythm: oscillations in the subthalamic nucleus-external globus pallidus network. Trends Neurosci. 25, 525–531.
Calabresi, P., Centonze, D., and Bernardi, G. (2000). Electrophysiology of dopamine in normal and denervated striatal neurons. Trends Neurosci. 23, S57–S63.
Calabresi, P., Mercuri, N. B., Sancesario, G., and Bernardi, G. (1993). Electrophysiology of dopamine-denervated striatal neurons. Implications for Parkinson’s disease. Brain 116(Pt 2), 433–452.
Calabresi, P., Picconi, B., Tozzi, A., and Di Filippo, M. (2007). Dopamine-mediated regulation of corticostriatal synaptic plasticity. Trends Neurosci. 30, 211–219.
Canteras, N. S., Shammah-Lagnado, S. J., Silva, B. A., and Ricardo, J. A. (1988). Somatosensory inputs to the subthalamic nucleus: a combined retrograde and anterograde horseradish peroxidase study in the rat. Brain Res. 458, 53–64.
Canteras, N. S., Shammah-Lagnado, S. J., Silva, B. A., and Ricardo, J. A. (1990). Afferent connections of the subthalamic nucleus: a combined retrograde and anterograde horseradish peroxidase study in the rat. Brain Res. 513, 43–59.
Chudasama, Y., Baunez, C., and Robbins, T. W. (2003). Functional disconnection of the medial prefrontal cortex and subthalamic nucleus in attentional performance: evidence for corticosubthalamic interaction. J. Neurosci. 23, 5477–5485.
Clarke, N. P., and Bolam, J. P. (1998). Distribution of glutamate receptor subunits at neurochemically characterized synapses in the entopeduncular nucleus and subthalamic nucleus of the rat. J. Comp. Neurol. 397, 403–420.
Coizet, V., Graham, J. H., Moss, J., Bolam, J. P., Savasta, M., Mchaffie, J. G., Redgrave, P., and Overton, P. G. (2009). Short-latency visual input to the subthalamic nucleus is provided by the midbrain superior colliculus. J. Neurosci. 29, 5701–5709.
Cowan, R. L., and Wilson, C. J. (1994). Spontaneous firing patterns and axonal projections of single corticostriatal neurons in the rat medial agranular cortex. J. Neurophysiol. 71, 17–32.
Cragg, S. J. (2003). Variable dopamine release probability and short-term plasticity between functional domains of the primate striatum. J. Neurosci. 23, 4378–4385.
Cragg, S. J., Baufreton, J., Xue, Y., Bolam, J. P., and Bevan, M. D. (2004). Synaptic release of dopamine in the subthalamic nucleus. Eur. J. Neurosci. 20, 1788–1802.
Delong, M., and Wichmann, T. (2009). Update on models of basal ganglia function and dysfunction. Parkinsonism Relat. Disord. 15(Suppl. 3), S237–S240.
Delong, M. R. (1990). Primate models of movement disorders of basal ganglia origin. Trends Neurosci. 13, 281–285.
Dias, R., Robbins, T. W., and Roberts, A. C. (1996). Dissociation in prefrontal cortex of affective and attentional shifts. Nature 380, 69–72.
Drouot, X., Oshino, S., Jarraya, B., Besret, L., Kishima, H., Remy, P., Dauguet, J., Lefaucheur, J. P., Dolle, F., Conde, F., Bottlaender, M., Peschanski, M., Keravel, Y., Hantraye, P., and Palfi, S. (2004). Functional recovery in a primate model of Parkinson’s disease following motor cortex stimulation. Neuron 44, 769–778.
Eagle, D. M., and Baunez, C. (2010). Is there an inhibitory-response-control system in the rat? Evidence from anatomical and pharmacological studies of behavioral inhibition. Neurosci. Biobehav. Rev. 34, 50–72.
Eagle, D. M., Baunez, C., Hutcheson, D. M., Lehmann, O., Shah, A. P., and Robbins, T. W. (2008). Stop-signal reaction-time task performance: role of prefrontal cortex and subthalamic nucleus. Cereb. Cortex 18, 178–188.
Feger, J., Bevan, M., and Crossman, A. R. (1994). The projections from the parafascicular thalamic nucleus to the subthalamic nucleus and the striatum arise from separate neuronal populations: a comparison with the corticostriatal and corticosubthalamic efferents in a retrograde fluorescent double-labelling study. Neuroscience 60, 125–132.
Flaherty, A. W., and Graybiel, A. M. (1991). Corticostriatal transformations in the primate somatosensory system. Projections from physiologically mapped body-part representations. J. Neurophysiol. 66, 1249–1263.
Flaherty, A. W., and Graybiel, A. M. (1993). Two input systems for body representations in the primate striatal matrix: experimental evidence in the squirrel monkey. J. Neurosci. 13, 1120–1137.
Florio, T., Di Loreto, S., Cerrito, F., and Scarnati, E. (1993). Influence of prelimbic and sensorimotor cortices on striatal neurons in the rat: electrophysiological evidence for converging inputs and the effects of 6-OHDA-induced degeneration of the substantia nigra. Brain Res. 619, 180–188.
Francois, C., Savy, C., Jan, C., Tande, D., Hirsch, E. C., and Yelnik, J. (2000). Dopaminergic innervation of the subthalamic nucleus in the normal state, in MPTP-treated monkeys, and in Parkinson’s disease patients. J. Comp. Neurol. 425, 121–129.
Fujimoto, K., and Kita, H. (1993). Response characteristics of subthalamic neurons to the stimulation of the sensorimotor cortex in the rat. Brain Res. 609, 185–192.
Fujiyama, F., Kuramoto, E., Okamoto, K., Hioki, H., Furuta, T., Zhou, L., Nomura, S., and Kaneko, T. (2004). Presynaptic localization of an AMPA-type glutamate receptor in corticostriatal and thalamostriatal axon terminals. Eur. J. Neurosci. 20, 3322–3330.
Galvan, A., Kuwajima, M., and Smith, Y. (2006). Glutamate and GABA receptors and transporters in the basal ganglia: what does their subsynaptic localization reveal about their function? Neuroscience 143, 351–375.
Giuffrida, R., Li Volsi, G., Maugeri, G., and Perciavalle, V. (1985). Influences of pyramidal tract on the subthalamic nucleus in the cat. Neurosci. Lett. 54, 231–235.
Goldman-Rakic, P. S., and Selemon, L. D. (1986). “Topography of corticostriatal projections in nonhuman primates and implications for functional parcellation of the neostriatum,” in Cerebral Cortex, eds E. G. Jones and A. Peters (New York: Plenum Press), 447–466.
Gradinaru, V., Mogri, M., Thompson, K. R., Henderson, J. M., and Deisseroth, K. (2009). Optical deconstruction of parkinsonian neural circuitry. Science 324, 354–359.
Graveland, G. A., Williams, R. S., and Difiglia, M. (1985). A Golgi study of the human neostriatum: neurons and afferent fibers. J. Comp. Neurol. 234, 317–333.
Hazrati, L. N., and Parent, A. (1992). Convergence of subthalamic and striatal efferents at pallidal level in primates: an anterograde double-labeling study with biocytin and PHA-L. Brain Res. 569, 336–340.
Hedreen, J. C. (1999). Tyrosine hydroxylase-immunoreactive elements in the human globus pallidus and subthalamic nucleus. J. Comp. Neurol. 409, 400–410.
Ingham, C. A., Hood, S. H., and Arbuthnott, G. W. (1989). Spine density on neostriatal neurones changes with 6-hydroxydopamine lesions and with age. Brain Res. 503, 334–338.
Ingham, C. A., Hood, S. H., Taggart, P., and Arbuthnott, G. W. (1998). Plasticity of synapses in the rat neostriatum after unilateral lesion of the nigrostriatal dopaminergic pathway. J. Neurosci. 18, 4732–4743.
Iwahori, N. (1978). A Golgi study on the subthalamic nucleus of the cat. J. Comp. Neurol. 182, 383–397.
Jinnai, K., and Matsuda, Y. (1979). Neurons of the motor cortex projecting commonly on the caudate nucleus and the lower brain stem in the cat. Neurosci. Lett. 13, 121–126.
Jones, E. G., Coulter, J. D., Burton, H., and Porter, R. (1977). Cells of origin and terminal distribution of corticostriatal fibers arising in the sensory-motor cortex of monkeys. J. Comp. Neurol. 173, 53–80.
Karachi, C., Yelnik, J., Tande, D., Tremblay, L., Hirsch, E. C., and Francois, C. (2005). The pallidosubthalamic projection: an anatomical substrate for nonmotor functions of the subthalamic nucleus in primates. Mov. Disord. 20, 172–180.
Kemp, J. M., and Powell, T. P. (1970). The cortico-striate projection in the monkey. Brain 93, 525–546.
Kemp, J. M., and Powell, T. P. (1971). The termination of fibres from the cerebral cortex and thalamus upon dendritic spines in the caudate nucleus: a study with the Golgi method. Philos. Trans. R. Soc. Lond. B Biol. Sci. 262, 429–439.
Kincaid, A. E., Zheng, T., and Wilson, C. J. (1998). Connectivity and convergence of single corticostriatal axons. J. Neurosci. 18, 4722–4731.
Kita, H. (1992). Responses of globus pallidus neurons to cortical stimulation: intracellular study in the rat. Brain Res. 589, 84–90.
Kitai, S. T., Kocsis, J. D., and Wood, J. (1976). Origin and characteristics of the cortico-caudate afferents: an anatomical and electrophysiological study. Brain Res. 118, 137–141.
Kolomiets, B. P., Deniau, J. M., Mailly, P., Menetrey, A., Glowinski, J., and Thierry, A. M. (2001). Segregation and convergence of information flow through the cortico-subthalamic pathways. J. Neurosci. 21, 5764–5772.
Kreitzer, A. C., and Malenka, R. C. (2008). Striatal plasticity and basal ganglia circuit function. Neuron 60, 543–554.
Kuwajima, M., Hall, R. A., Aiba, A., and Smith, Y. (2004). Subcellular and subsynaptic localization of group I metabotropic glutamate receptors in the monkey subthalamic nucleus. J. Comp. Neurol. 474, 589–602.
Landry, P., Wilson, C. J., and Kitai, S. T. (1984). Morphological and electrophysiological characteristics of pyramidal tract neurons in the rat. Exp. Brain Res. 57, 177–190.
Lavoie, B., Smith, Y., and Parent, A. (1989). Dopaminergic innervation of the basal ganglia in the squirrel monkey as revealed by tyrosine hydroxylase immunohistochemistry. J. Comp. Neurol. 289, 36–52.
Lei, W., Jiao, Y., Del Mar, N., and Reiner, A. (2004). Evidence for differential cortical input to direct pathway versus indirect pathway striatal projection neurons in rats. J. Neurosci. 24, 8289–8299.
Levesque, M., Charara, A., Gagnon, S., Parent, A., and Deschenes, M. (1996a). Corticostriatal projections from layer V cells in rat are collaterals of long-range corticofugal axons. Brain Res. 709, 311–315.
Levesque, M., Gagnon, S., Parent, A., and Deschenes, M. (1996b). Axonal arborizations of corticostriatal and corticothalamic fibers arising from the second somatosensory area in the rat. Cereb. Cortex 6, 759–770.
Levesque, M., and Parent, A. (1998). Axonal arborization of corticostriatal and corticothalamic fibers arising from prelimbic cortex in the rat. Cereb. Cortex 8, 602–613.
Li, S., Arbuthnott, G. W., Jutras, M. J., Goldberg, J. A., and Jaeger, D. (2007). Resonant antidromic cortical circuit activation as a consequence of high-frequency subthalamic deep-brain stimulation. J. Neurophysiol. 98, 3525–3537.
Liang, L., Delong, M. R., and Papa, S. M. (2008). Inversion of dopamine responses in striatal medium spiny neurons and involuntary movements. J. Neurosci. 28, 7537–7547.
Magill, P. J., Sharott, A., Bevan, M. D., Brown, P., and Bolam, J. P. (2004). Synchronous unit activity and local field potentials evoked in the subthalamic nucleus by cortical stimulation. J. Neurophysiol. 92, 700–714.
Mahon, S., Deniau, J. M., and Charpier, S. (2001). Relationship between EEG potentials and intracellular activity of striatal and cortico-striatal neurons: an in vivo study under different anesthetics. Cereb. Cortex 11, 360–373.
Mathai, A., Pare, J. F., Jenkins, S., and Smith, Y. (2010). “Glutamatergic inputs to the subthalamic nucleus: a quantitative analysis of the synaptic microcircuitry of vGluT1- and vGluT2-containing terminals in normal and Parkinsonian nonhuman primates,” in Xth Triennial Meeting of the International Basal Ganglia Society, Long Branch, NJ.
Maurice, N., Deniau, J. M., Glowinski, J., and Thierry, A. M. (1999). Relationships between the prefrontal cortex and the basal ganglia in the rat: physiology of the cortico-nigral circuits. J. Neurosci. 19, 4674–4681.
Mcgeorge, A. J., and Faull, R. L. (1989). The organization of the projection from the cerebral cortex to the striatum in the rat. Neuroscience 29, 503–537.
Mediratta, N. K., and Nicoll, J. A. (1983). Conduction velocities of corticospinal axons in the rat studied by recording cortical antidromic responses. J. Physiol. (Lond.) 336, 545–561.
Mink, J. W. (1996). The basal ganglia: focused selection and inhibition of competing motor programs. Prog. Neurobiol. 50, 381–425.
Monakow, K. H., Akert, K., and Kunzle, H. (1978). Projections of the precentral motor cortex and other cortical areas of the frontal lobe to the subthalamic nucleus in the monkey. Exp. Brain Res. 33, 395–403.
Morishima, M., and Kawaguchi, Y. (2006). Recurrent connection patterns of corticostriatal pyramidal cells in frontal cortex. J. Neurosci. 26, 4394–4405.
Nambu, A., Takada, M., Inase, M., and Tokuno, H. (1996). Dual somatotopical representations in the primate subthalamic nucleus: evidence for ordered but reversed body-map transformations from the primary motor cortex and the supplementary motor area. J. Neurosci. 16, 2671–2683.
Nambu, A., Tokuno, H., Hamada, I., Kita, H., Imanishi, M., Akazawa, T., Ikeuchi, Y., and Hasegawa, N. (2000). Excitatory cortical inputs to pallidal neurons via the subthalamic nucleus in the monkey. J. Neurophysiol. 84, 289–300.
Nambu, A., Tokuno, H., Inase, M., and Takada, M. (1997). Corticosubthalamic input zones from forelimb representations of the dorsal and ventral divisions of the premotor cortex in the macaque monkey: comparison with the input zones from the primary motor cortex and the supplementary motor area. Neurosci. Lett. 239, 13–16.
Nambu, A., Tokuno, H., and Takada, M. (2002). Functional significance of the cortico-subthalamo-pallidal “hyperdirect” pathway. Neurosci. Res. 43, 111–117.
Nicola, S. M., Surmeier, J., and Malenka, R. C. (2000). Dopaminergic modulation of neuronal excitability in the striatum and nucleus accumbens. Annu. Rev. Neurosci. 23, 185–215.
Ohye, C., Le Gayader, C., and Feger, J. (1976). Responses of subthalamic and pallidal neurons to striatal stimulation: an extracellular study on awake monkeys. Brain Res. 111, 241–252.
Oka, H. (1980). Organization of the cortico-caudate projections. A horseradish peroxidase study in the cat. Exp. Brain Res. 40, 203–208.
Onn, S. P., and Grace, A. A. (1999). Alterations in electrophysiological activity and dye coupling of striatal spiny and aspiny neurons in dopamine-denervated rat striatum recorded in vivo. Synapse 33, 1–15.
Onn, S. P., West, A. R., and Grace, A. A. (2000). Dopamine-mediated regulation of striatal neuronal and network interactions. Trends Neurosci. 23, S48–S56.
Pang, Z., Ling, G. Y., Gajendiran, M., and Xu, Z. C. (2001). Enhanced excitatory synaptic transmission in spiny neurons of rat striatum after unilateral dopamine denervation. Neurosci. Lett. 308, 201–205.
Paquet, M., and Smith, Y. (2003). Group I metabotropic glutamate receptors in the monkey striatum: subsynaptic association with glutamatergic and dopaminergic afferents. J. Neurosci. 23, 7659–7669.
Paradiso, G., Saint-Cyr, J. A., Lozano, A. M., Lang, A. E., and Chen, R. (2003). Involvement of the human subthalamic nucleus in movement preparation. Neurology 61, 1538–1545.
Parent, A., Charara, A., and Pinault, D. (1995). Single striatofugal axons arborizing in both pallidal segments and in the substantia nigra in primates. Brain Res. 698, 280–284.
Parent, A., and Hazrati, L. N. (1995). Functional anatomy of the basal ganglia. I. The cortico-basal ganglia-thalamo-cortical loop. Brain Res. Brain Res. Rev. 20, 91–127.
Parent, M., and Parent, A. (2006). Single-axon tracing study of corticostriatal projections arising from primary motor cortex in primates. J. Comp. Neurol. 496, 202–213.
Parthasarathy, H. B., Schall, J. D., and Graybiel, A. M. (1992). Distributed but convergent ordering of corticostriatal projections: analysis of the frontal eye field and the supplementary eye field in the macaque monkey. J. Neurosci. 12, 4468–4488.
Pawlak, V., and Kerr, J. N. (2008). Dopamine receptor activation is required for corticostriatal spike-timing-dependent plasticity. J. Neurosci. 28, 2435–2446.
Paz, J. T., Deniau, J. M., and Charpier, S. (2005). Rhythmic bursting in the cortico-subthalamo-pallidal network during spontaneous genetically determined spike and wave discharges. J. Neurosci. 25, 2092–2101.
Picconi, B., Centonze, D., Hakansson, K., Bernardi, G., Greengard, P., Fisone, G., Cenci, M. A., and Calabresi, P. (2003). Loss of bidirectional striatal synaptic plasticity in L-DOPA-induced dyskinesia. Nat. Neurosci. 6, 501–506.
Raju, D. V., Ahern, T. H., Shah, D. J., Wright, T. M., Standaert, D. G., Hall, R. A., and Smith, Y. (2008). Differential synaptic plasticity of the corticostriatal and thalamostriatal systems in an MPTP-treated monkey model of parkinsonism. Eur. J. Neurosci. 27, 1647–1658.
Raju, D. V., Shah, D. J., Wright, T. M., Hall, R. A., and Smith, Y. (2006). Differential synaptology of vGluT2-containing thalamostriatal afferents between the patch and matrix compartments in rats. J. Comp. Neurol. 499, 231–243.
Reiner, A., Jiao, Y., Del Mar, N., Laverghetta, A. V., and Lei, W. L. (2003). Differential morphology of pyramidal tract-type and intratelencephalically projecting-type corticostriatal neurons and their intrastriatal terminals in rats. J. Comp. Neurol. 457, 420–440.
Reynolds, J. N., and Wickens, J. R. (2002). Dopamine-dependent plasticity of corticostriatal synapses. Neural Netw. 15, 507–521.
Rommelfanger, K. S., and Wichmann, T. (2010). Extrastriatal dopaminergic circuits of the basal ganglia. Front. Neuroanat. 4:139. doi: 10.3389/fnana.2010.00139
Rouzaire-Dubois, B., and Scarnati, E. (1985). Bilateral corticosubthalamic nucleus projections: an electrophysiological study in rats with chronic cerebral lesions. Neuroscience 15, 69–79.
Royce, G. J. (1982). Laminar origin of cortical neurons which project upon the caudate nucleus: a horseradish peroxidase investigation in the cat. J. Comp. Neurol. 205, 8–29.
Sato, F., Parent, M., Levesque, M., and Parent, A. (2000). Axonal branching pattern of neurons of the subthalamic nucleus in primates. J. Comp. Neurol. 424, 142–152.
Selemon, L. D., and Goldman-Rakic, P. S. (1985). Longitudinal topography and interdigitation of corticostriatal projections in the rhesus monkey. J. Neurosci. 5, 776–794.
Shink, E., Bevan, M. D., Bolam, J. P., and Smith, Y. (1996). The subthalamic nucleus and the external pallidum: two tightly interconnected structures that control the output of the basal ganglia in the monkey. Neuroscience 73, 335–357.
Slaght, S. J., Paz, T., Chavez, M., Deniau, J. M., Mahon, S., and Charpier, S. (2004). On the activity of the corticostriatal networks during spike-and-wave discharges in a genetic model of absence epilepsy. J. Neurosci. 24, 6816–6825.
Smith, Y. (2011). “Functional anatomy of the motor and non-motor circuitry of the basal ganglia,” in Parkinson’s Disease: Non-Motor and Non-Dopaminergic Features, 1st Edn, eds C. W. Olanow, F. Stocchi and A. Lang (Oxford: Wiley-Blackwell), 32–55.
Smith, Y., Bevan, M. D., Shink, E., and Bolam, J. P. (1998). Microcircuitry of the direct and indirect pathways of the basal ganglia. Neuroscience 86, 353–387.
Smith, Y., Bolam, J. P., and Von Krosigk, M. (1990). Topographical and synaptic organization of the GABA-containing pallidosubthalamic projection in the rat. Eur. J. Neurosci. 2, 500–511.
Smith, Y., and Kieval, J. Z. (2000). Anatomy of the dopamine system in the basal ganglia. Trends Neurosci. 23, S28–S33.
Smith, Y., and Villalba, R. (2008). Striatal and extrastriatal dopamine in the basal ganglia: an overview of its anatomical organization in normal and Parkinsonian brains. Mov. Disord. 23(Suppl. 3), S534–S547.
Stephens, B., Mueller, A. J., Shering, A. F., Hood, S. H., Taggart, P., Arbuthnott, G. W., Bell, J. E., Kilford, L., Kingsbury, A. E., Daniel, S. E., and Ingham, C. A. (2005). Evidence of a breakdown of corticostriatal connections in Parkinson’s disease. Neuroscience 132, 741–754.
Strafella, A. P., Ko, J. H., Grant, J., Fraraccio, M., and Monchi, O. (2005). Corticostriatal functional interactions in Parkinson’s disease: a rTMS/[11C]raclopride PET study. Eur. J. Neurosci. 22, 2946–2952.
Surmeier, D. J., Ding, J., Day, M., Wang, Z., and Shen, W. (2007). D1 and D2 dopamine-receptor modulation of striatal glutamatergic signaling in striatal medium spiny neurons. Trends Neurosci. 30, 228–235.
Surmeier, D. J., Plotkin, J., and Shen, W. (2009). Dopamine and synaptic plasticity in dorsal striatal circuits controlling action selection. Curr. Opin. Neurobiol. 19, 621–628.
Takada, M., Tokuno, H., Hamada, I., Inase, M., Ito, Y., Imanishi, M., Hasegawa, N., Akazawa, T., Hatanaka, N., and Nambu, A. (2001). Organization of inputs from cingulate motor areas to basal ganglia in macaque monkey. Eur. J. Neurosci. 14, 1633–1650.
Tanaka, D. Jr. (1987). Differential laminar distribution of corticostriatal neurons in the prefrontal and pericruciate gyri of the dog. J. Neurosci. 7, 4095–4106.
Temel, Y., Kessels, A., Tan, S., Topdag, A., Boon, P., and Visser-Vandewalle, V. (2006). Behavioural changes after bilateral subthalamic stimulation in advanced Parkinson disease: a systematic review. Parkinsonism Relat. Disord. 12, 265–272.
Turner, R. S., and Delong, M. R. (2000). Corticostriatal activity in primary motor cortex of the macaque. J. Neurosci. 20, 7096–7108.
Veening, J. G., Cornelissen, F. M., and Lieven, P. A. (1980). The topical organization of the afferents to the caudatoputamen of the rat. A horseradish peroxidase study. Neuroscience 5, 1253–1268.
Villalba, R. M., Lee, H., and Smith, Y. (2009). Dopaminergic denervation and spine loss in the striatum of MPTP-treated monkeys. Exp. Neurol. 215, 220–227.
Villalba, R. M., and Smith, Y. (2010). Striatal spine plasticity in Parkinson’s disease. Front. Neuroanat. 4:133. doi: 10.3389/fnana.2010.00133
Wichmann, T., Bergman, H., and Delong, M. R. (1994). The primate subthalamic nucleus. I. Functional properties in intact animals. J. Neurophysiol. 72, 494–506.
Wichmann, T., and Delong, M. R. (1996). Functional and pathophysiological models of the basal ganglia. Curr. Opin. Neurobiol. 6, 751–758.
Wilson, C. J. (1987). Morphology and synaptic connections of crossed corticostriatal neurons in the rat. J. Comp. Neurol. 263, 567–580.
Wright, A. K., Norrie, L., Ingham, C. A., Hutton, E. A., and Arbuthnott, G. W. (1999). Double anterograde tracing of outputs from adjacent “barrel columns” of rat somatosensory cortex. Neostriatal projection patterns and terminal ultrastructure. Neuroscience 88, 119–133.
Wright, A. K., Ramanathan, S., and Arbuthnott, G. W. (2001). Identification of the source of the bilateral projection system from cortex to somatosensory neostriatum and an exploration of its physiological actions. Neuroscience 103, 87–96.
Xu, Z. C., Wilson, C. J., and Emson, P. C. (1989). Restoration of the corticostriatal projection in rat neostriatal grafts: electron microscopic analysis. Neuroscience 29, 539–550.
Yelnik, J., and Percheron, G. (1979). Subthalamic neurons in primates: a quantitative and comparative analysis. Neuroscience 4, 1717–1743.
Yeterian, E. H., and Van Hoesen, G. W. (1978). Cortico-striate projections in the rhesus monkey: the organization of certain cortico-caudate connections. Brain Res. 139, 43–63.
Zaja-Milatovic, S., Milatovic, D., Schantz, A. M., Zhang, J., Montine, K. S., Samii, A., Deutch, A. Y., and Montine, T. J. (2005). Dendritic degeneration in neostriatal medium spiny neurons in Parkinson disease. Neurology 64, 545–547.
Keywords: subthalamic nucleus, striatum, hyperdirect, Parkinson’s disease, basal ganglia, cerebral cortex, monkey, deep brain stimulation
Citation: Mathai A and Smith Y (2011) The corticostriatal and corticosubthalamic pathways: two entries, one target. So what? Front. Syst. Neurosci. 5:64. doi: 10.3389/fnsys.2011.00064
Received: 28 April 2011; Paper pending published: 13 June 2011;
Accepted: 21 July 2011; Published online: 01 August 2011.
Edited by:
Charles J. Wilson, University of Texas at San Antonio, USAReviewed by:
Anton Reiner, University of Tennessee Health Science Center, USAPeter J. Magill, University of Oxford, UK
Copyright: © 2011 Mathai and Smith. This is an open-access article subject to a non-exclusive license between the authors and Frontiers Media SA, which permits use, distribution and reproduction in other forums, provided the original authors and source are credited and other Frontiers conditions are complied with.
*Correspondence: Yoland Smith, Yerkes National Primate Research Center, 954 Gatewood Road NE, Atlanta, GA 30329, USA. e-mail: ysmit01@emory.edu