The effect of severe intensity bouts on muscle oxygen saturation responses in trained cyclists
- 1Environmental Physiology Laboratory, The University of British Columbia, School of Kinesiology, Vancouver, BC, Canada
- 2Department of Biomedical Physiology and Kinesiology and Sports Analytics Group, Simon Fraser University, Burnaby, Canada
- 3Deptartment of Physical Therapy, The University of British Columbia, Vancouver, BC, Canada
- 4Centre for Heart Lung Innovation, Providence Research, The University of British Columbia and St. Paul's Hospital, Vancouver, BC, Canada
- 5Department of Family Practice, Vancouver Whitecaps FC, Vancouver, BC, Canada
- 6Division of Sports Medicine, The University of British Columbia, Vancouver, BC, Canada
Near-infrared spectroscopy (NIRS) quantifies muscle oxygenation (SmO2) during exercise. Muscle oxygenation response to self-paced, severe-intensity cycling remains unclear. Observing SmO2 can provide cycling professionals with the ability to assess muscular response, helping optimize decision-making. We aimed to describe the effect of self-paced severe intensity bouts on SmO2, measured noninvasively by a wearable NIRS sensor on the vastus lateralis (VL) muscle, and examine its reliability. We hypothesized a greater desaturation response with each bout, whereas, between trials, good reliability would be observed. Fourteen recreationally trained, and trained cyclists completed a ramp test to determine the power output (PO) at the respiratory compensation point (RCP). Athletes completed two subsequent visits of 50-minute sessions that included four severe-intensity bouts done at 5% above RCP PO. Muscle oxygenation in the VL was monitored using a wearable NIRS device. Measures included mean PO, heart-rate (HR), cadence, and SmO2 at bout onset, during work (work SmO2), and ΔSmO2. The bouts were compared using a one-way repeated measures ANOVA. For significant differences, a Fisher's least square difference post-hoc analysis was used. A two-way repeated measures ANOVA was used using trial and bout as main factors. Intraclass correlations (ICC) were used to quantify relative reliability for mean work, and standard error of the measurement (SEM) was used to quantify absolute agreement of mean work SmO2. Both PO and cadence showed no effect of bout or trial. Heart-rate at bout 2 (168 ± 8 bpm) and 4 (170 ± 7 bpm) were higher than bout 1 (160 ± 6 bpm). Onset SmO2 (%) response significantly increased in the final two bouts of the session. Mean work SmO2 increased across bouts, with the highest value displayed in bout 4 (36 ± 22%). ΔSmO2 showed a smaller desaturation response during bout 4 (27 ± 10%) compared to bout 3 (31 ± 10%). Mean work SmO2 ICC showed good reliability (ICC = 0.87), and SEM was 12% (CI 9-15%). We concluded that a non-invasive, affordable, wearable NIRS sensor demonstrated the heterogeneous muscle oxygenation response during severe intensity cycling bouts with good reliability in trained cyclists.
1. Introduction
Numerous technological advancements in the sport of cycling provide a unique platform for gathering valuable input regarding the effects of exercise intensity on systemic responses, both in the field and in real-time (1). Such advancements primarily focus on measuring external load during training via onboard power meters, which measure power output (PO) directly from the bicycle drivetrain. Additionally, heart rate (HR) measurements are equally popular and provide a useful signal for cardiovascular responses and adaptations (2). Understanding this relationship solely through the lens of HR response provides little information about local intramuscular responses during exercise, especially under severe intensity exercise loads where a non-steady state is expected. Understanding muscle metabolic activity during severe intensity cycling exercise, in conjunction with PO and HR measurements, can provide critical information concerning muscular limitations, which can aid in prescribing specific interventions to optimize cycling performance.
Muscle oxygenation via near-infrared spectroscopy (NIRS) has been investigated extensively at a range of exercise intensities and activity types (3). With its rise in popularity, NIRS technology has generated good reproducible results under controlled conditions, primarily with the use of stationary, expensive, laser-based spectrometers (4–7). More recently, continuous-wave light-emitting diode spectrometers offer the possibility of integrating NIRS into portable, wearable units (3). Such designs introduced the possibility of assessing the muscle oxygenation response in training and competition environments, at a greatly reduced cost (3, 8).
Near-infrared spectroscopy is sensitive to the reflectivity of hemoglobin and myoglobin in microcirculation and muscle tissue, detecting their oxygenated (O2HbMb) and deoxygenated (HHbMb) forms and their sum total (tHbMb) (9). The relative portion of O2Hb + Mb from tHb + Mb is used to estimate percent muscle oxygen saturation (SmO2). The latter is commonly used by commercially available wearable NIRS manufacturers (10, 11). SmO2 tends to decrease with increasing exercise intensity (12). Multiple studies that measure muscle oxygenation during exercise to task intolerance report attainment of an SmO2 nadir at the primary locomotor muscles (13–16). During constant workload exercise, the profile of SmO2 is generally the inverse of pulmonary oxygen consumption (17). During severe intensity exercise, we might then expect to see progressive deoxygenation in the SmO2 response, similar to the V˙O2 slow component (17, 18). The ability to display local SmO2 response during submaximal, severe intensities in real-time can assist athletes and performance specialists to track muscle oxygen utilization relative to workload and optimize pacing strategy accordingly. Observing this effect during self-paced, severe intensity bouts during cycling remains undescribed.
Currently, test-retest reliability of NIRS has been investigated at rest and in various exercise contexts (5, 10, 13). For wearable NIRS, assessment of its reliability for measuring muscle oxygenation during severe intensity exercise in field-relevant training environments is needed to reveal possible measurement limitations prior to deployment in the field. Accordingly, the purpose of this study was two-fold: 1) describe the effect of self-paced, severe-intensity cycling bouts on SmO2 of the vastus lateralis (VL) muscle and 2) quantify the test-retest reliability of SmO2 between cycling exercise sessions. Within session, we hypothesized a decreased SmO2 with each bout number, whereas we hypothesized good reliability for SmO2.
2. Materials and methods
2.1. Subjects
Fourteen trained, and recreationally trained cyclists (7 females & 7 males, 74.1 ± 10.5 kg, 32.1 ± 7.6 years of age, 170.0 ± 11.0 cm, 11.3 ± 5.4 mm VL skinfold thickness, and 55.0 ± 9.1 ml·kg·min−1 maximum oxygen uptake) volunteered and provided written informed consent to participate in the study (19). To obtain sufficient power of β = 0.8 with α = 0.05, an a priori sample size calculation was made in G*Power software (version 3.1.9.7, Kiel, Germany) using previously reported data from other groups that compared SmO2 values within and between sessions during ramp incremental tests and severe intensity efforts (16, 20–23). This study was approved by the research ethics committee of The University of British Columbia and was conducted in accordance with principles established in the Declaration of Helsinki, except for registration in a database.
2.2. Experimental design
2.2.1. Incremental ramp cycling exercise
The study consisted of three visits. The protocol and method used to estimate PO at the respiratory compensation point (RCP) during the first visit was previously described (15), and included an incremental ramp cycling test from rest to task intolerance. Participants completed the protocol on an electronically controlled, stationary bicycle trainer (KICKR, Wahoo Fitness Inc., Atlanta, GA, USA) using their own bicycle at a gear ratio that simulated their regular indoor training. The ramp rate increased by 1 W every 2 s (30 W·min−1), with task intolerance determined as the point at which the participant's self-selected cadence went down by more than 10 revolutions per minute (rpm). Resistance was controlled in ergometer mode using PerfPRO Studio Software© (Hartware Technologies, Rockford, MI, USA). During the exercise test, ventilation (Ve), pulmonary oxygen (V˙O2), and carbon dioxide uptake (V˙CO2) were measured with an open-circuit expired-gas analysis system (TrueOne 2400; ParvoMedics, Inc, Sandy, UT). Following the test, the PO at the RCP was estimated, correcting for individual muscle to lung transit time and used to calculate the PO during the follow-up sessions (105% of RCP PO) (15).
Pulmonary oxygen uptake (V˙O2) was measured with an open-circuit expired-gas analysis system (TrueOne 2400; ParvoMedics, Inc, Sandy, UT). V˙O2 data were averaged to 15 s and interpolated to 1 Hz for analysis. V˙O2peak was considered the highest average 30-second measurement. The RCP was determined at the point of deflection of V˙E relative to V˙CO2, and the second deflection of V˙E relative to V˙O2 (24, 25).
An individual mean response time (MRT) representing the delay between muscular metabolic activity and pulmonary response was determined using a recently described protocol (26, 27). Briefly, the subjects performed a baseline warm-up for 6 min at a moderate PO of either 110 W (females) or 140 W (males). Average baseline V˙O2 was determined from the final 2 min of the baseline step. The ramp exercise test began with 4 min at 70 W (females) or 100 W (males), before the continuous ramp commenced at 1 W per 2 s. The subject's V˙O2 response during the ramp test was compared to their average baseline V˙O2. The difference in the instantaneous PO that elicited the same V˙O2 response was used to determine the MRT in watts and in seconds. The MRT was then used to shift PO relative to V˙O2 for estimation of the PO that elicited RCP (15).
2.2.2. Severe-intensity interval exercise
The second and third visits included the same ergometer setup as described in the initial visit, and the protocol is shown in Figure 1. The 2nd and 3rd visits are identical and used to determine repeatability. To ensure participants rode within their individual severe intensity domain, bouts were done at 5% above estimated RCP PO from the first visitation (14). The duration of the severe intensity bout was 4 min. The time of the step was selected to allow for a physiological pseudo steady state to occur (28, 29). During both sessions, an initial 10-minute warm-up at 40% of RCP PO was used. Immediately after the warmup, a zero-offset calibration was conducted to ensure the trainer provided accurate resistance. During the first interval session, participants were asked to pedal at a self-selected cadence. Participants were instructed to keep the same cadence (± 5 rpm) and gear ratio to ensure the same workload for the subsequent bouts across both sessions. The use of self-pacing with a target PO, rather than controlling the resistance of the cycling ergometer, was to simulate real-world training conditions. Between bouts, one-minute passive recovery was performed, followed by five minutes at 50% RCP PO. The electronic trainer was set to simulate a gradient of 1%. Measures of PO were measured in all participants, whereas HR and cadence were measure in only 7 participants. These variables were measured continuously using the stationary bicycle trainer, the participant cadence sensor, and a HR monitor (HRM1G; Garmin Ltd., Olathe, KS, USA), respectively.
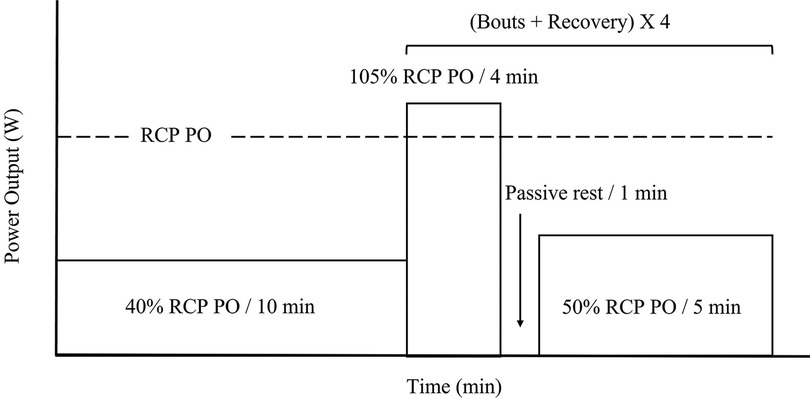
Figure 1. Severe intensity interval exercise protocol. Percent power output (PO) is calculated from PO detected at respiratory compensation point (RCP) (dotted line) from initial ramp test. Ten-minutes warm up at 40% RCP PO, followed by four severe intensity bouts of four minutes at 105% of RCP PO, one minute passive rest, and five minutes active recovery at 50% RCP PO.
2.3. NIRS
Two wearable NIRS sensors (Moxy Monitor, Fortiori Design LLC., Hutchinson, USA) were used during the test. The Moxy monitor employs four wavelengths of near-infrared light (680, 720, 760, and 800 nm), with source detector separation of 12.5 and 25 mm (10). The sensors were placed on the right and left VL, and the right side was used for analysis and the left was used in case of right sensor failure. In all but one participant, the right sensor was used. The anatomical location on the VL was 1/3 the distance from the proximal pole of the patella to the greater trochanter. Left and right sensors were held in place by the participants' elastic cycling shorts, and both sensors were covered using a light shield supplied by the manufacturer to minimize ultraviolet light interference.
During instrumentation, skinfold thickness was measured and recorded from the right VL with a Harpenden skinfold caliper (Creative Health, Dallas, USA). According to the manufacturer, the Moxy sensor does not require calibration (10, 11). Prior to each trial, the sensor was charged, and both the emitter and receiving optodes were cleaned.
2.4. Data analysis
The Moxy sensor provides measures of total heme concentration (tHb + Mb in arbitrary units), and muscle O2 saturation (SmO2 as a percent). The SmO2 signal was used as the primary output variable in this study (10, 11, 21). SmO2 was measured every 2 s (0.5 Hz) and raw data were smoothed to 5-second moving averages as per manufacturer default settings. The signal profile was assessed at two points during each of the four severe intensity bouts completed in each session: peak SmO2 value at exercise onset and mean 30-sec SmO2 at the end of the work phase (work SmO2). Additionally, the amplitude of deoxygenation during work was analysed as the change from onset to work SmO2 (ΔSmO2).
Data acquisition was done in a training analysis software WKO5 (TrainingPeaks, LLC, Boulder, CO, USA) to find the relevant values at the two phases of each interval. Onset was calculated as the highest SmO2 value during the first 60 s of each severe intensity bout. Work SmO2 was measured as the mean SmO2 of the final two minutes of each bout (Figure 2). The ΔSmO2 was calculated from the difference between maximum SmO2 value at exercise onset to the final 30 s average SmO2 of each bout (Figure 2).
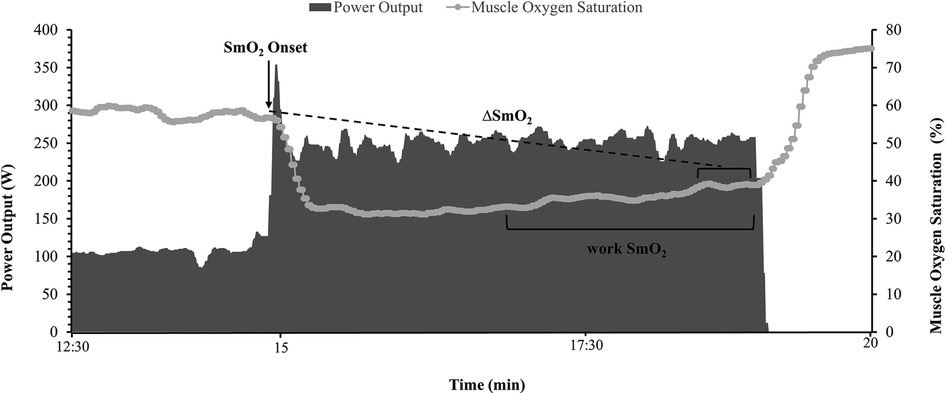
Figure 2. Illustration of muscle oxygen saturation (SmO2) at exercise onset (black arrow), mean work phase SmO2 during the final two minutes of the severe intensity bout, and ΔSmO2 calculation of the difference between SmO2 at bout onset and the 30s average SmO2 at the end of the effort (dotted line).
2.5. Statistical analysis
Data analysis was performed using GraphPad statistical software (GraphPad Software Inc., CA, USA), and IBM SPSS Statistics version 28 (IBM Inc., NY, USA). After initially recruiting only 7 males for the study, a decision was made to recruit more females for the study to both to improve applicability across sex and increase total sample size. One-way repeated measures ANOVAs were used to analyze the effect of bout on all outcome variables. The alpha level was set at 0.05. A Fisher's, least square difference, post-hoc test was used if a main effect was detected. Two-way repeated measures ANOVAs were used to analyze the interaction of trial and bout on all outcome variables. The alpha level was set at 0.05. A Bonferroni post-hoc analysis was used if a main effect for an interaction was detected. Normality was assessed by Shapiro-Wilk tests.
Previous studies reported the reliability of work-phase muscle oxygenation response (5, 10). As such, our reliability analysis used mean work SmO2 (%) as a primary outcome variable. Relative reliability was quantified as intraclass correlation coefficient (ICC3,k) (30, 31). Values of < 0.5 (poor), 0.5–75 (moderate), 0.75–0.9 (good), and > 0.9 (excellent) reliability were used (32). Absolute reliability evaluates the consistency of repeated measurements (repeatability) within a single individual. Absolute reliability was quantified as the standard error of the measurement (SEM) (30, 31, 33).
3. Results
3.1. Within session analysis
Within session means for PO, HR, cadence, onset, work SmO2, and ΔSmO2 are presented in Table 1. A representative dataset for SmO2 during the severe intensity interval exercise is presented in Figure 3. For HR and cadence, data was available for only seven participants. One-way repeated measures ANOVAs were performed to detect an effect of bout on PO, HR, and cadence. No effect of bout on PO or cadence was detected (Figures 4A,C). A main effect of bout was found for HR (Figure 4B); mean HR at bouts 2 (168 ± 8 bpm), and 4 (170 ± 7 bpm) were significantly different from bout 1 (160 ± 6 bpm) (p < 0.05).
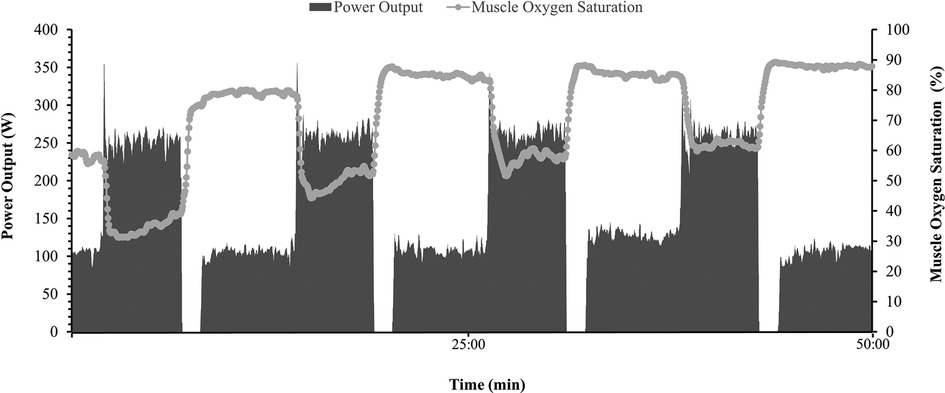
Figure 3. Representative dataset (n = 1) during a 50 min mixed intensity session. Power output (W) is represented on right Y axis by shaded bars, Heart rate (bpm) is presented on left Y axis by solid gray line, and muscle oxygen saturation (%) is displayed on left Y axis by gray dots and line.
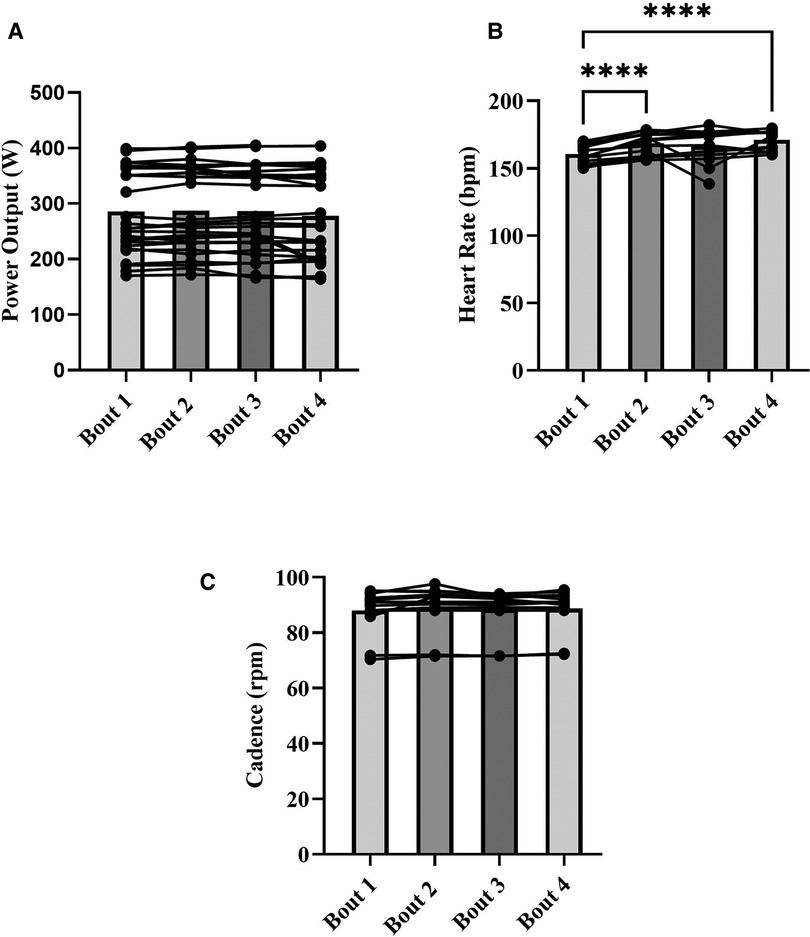
Figure 4. Means for power output (W) (A), heart rate (bpm) (B), and cadence (rpm) (C) for each severe intensity interval (bars), with representation of individual responses across the four bouts from both sessions (dots + lines). *p < 0.05, ****p < 0.0001.
For NIRS outcomes, one-way repeated measures ANOVAs were performed to detect an effect of bout on onset (%), work SmO2 (%), and ΔSmO2 (%). A main effect of bout was detected for all NIRS outcome variables. For onset, bout 1 (55 ± 15%) and 2 (58 ± 18%) were not different from each other but were different from bouts 3 (62 ± 17) and 4 (62 ± 18) (p < 0.05) (Figure 5A). Significant differences in work SmO2 were detected across all bouts (p < 0.05), with an increasing trend from 30 ± 12% in bout 1 to 37 ± 21% in bout 4 (Figure 5B). A significant difference was detected in ΔSmO2 between bout 3 (31 ± 10%) and bout 4 (27 ± 10%) (p < 0.05) (Figure 5C).
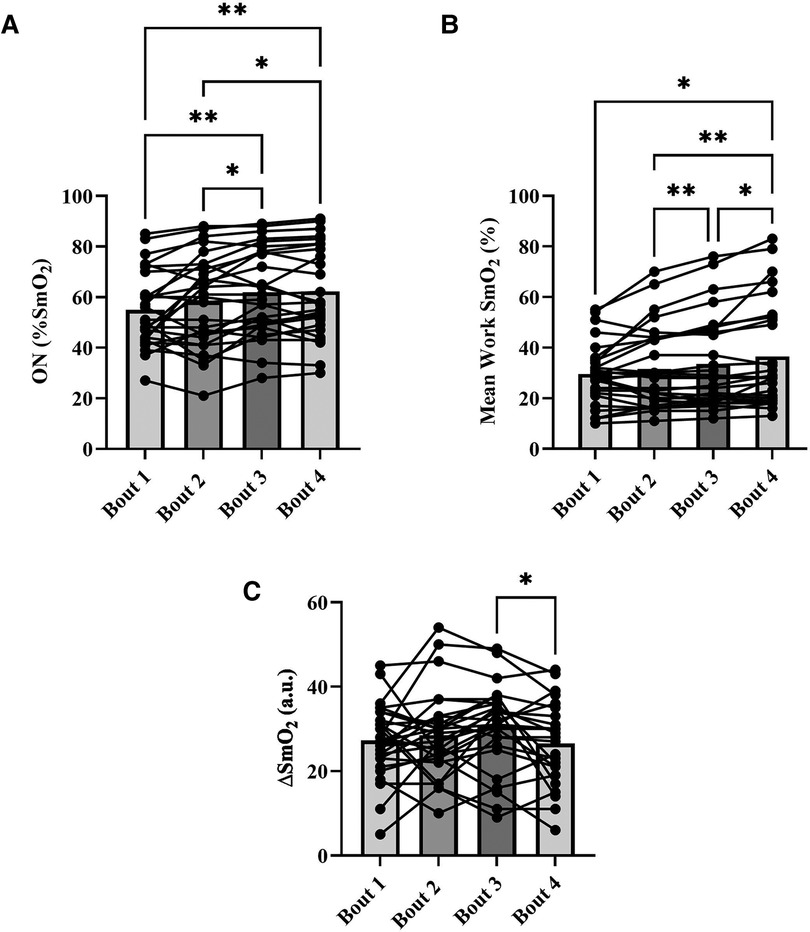
Figure 5. Means for muscle oxygen saturation (SmO2) at bout onset (%) (A), mean work SmO2 (%) (B), and mean SmO2 amplitude (ΔSmO2) (%) (C), for each severe intensity bout (bars), with representation of individual responses across the four bouts from both sessions (dots + lines). *p < 0.05, **p < 0.01.
3.2. Reliability analysis
Means for PO, HR, cadence, onset SmO2, work SmO2, and ΔSmO2, as well as relative and absolute agreements between sessions are presented in Table 2. Two-way repeated measures ANOVAs were used to detect a main effect for an interaction between trial and bout on all outcome variables. For PO, a main effect for an interaction was detected (F = 3.3, p < 0.05), with no main effect of either trial or bout. For cadence, no main effect for an interaction was detected, with no main effect of either trial or bout. For HR, a simple main effect of bout was found (F = 26.6, p < 0.01), with no main effect for an interaction, or for trial. For the effect of bout, a post-hoc analysis resulted in a significant difference between bouts 1 (161 ± 9, and 160 ± 8 bpm) and the remaining bouts (p < 0.05).
For NIRS outcome variables, a simple main effect of bout on onset SmO2 was found (F = 6.2, p < 0.01), with no main effect for an interaction, or for trial. For the effect of bout, a post-hoc analysis resulted in a significant difference between bouts 1 (54.0 ± 16.0, and 56.0 ± 15.2%) and bouts 4 (62.3 ± 19, and 60 ± 19%) (p < 0.05). For work SmO2, a main effect for an interaction was detected (F = 4.2, p < 0.05). Simple main effects analysis showed that bout did have a statistically significant effect on work SmO2 (F = 5.5, p < 0.01). Post-hoc analysis detected a significant difference between bouts 2 (32.0 ± 18.0, and 29.1 ± 12.3%) and bouts 4 (36.0 ± 20, and 41.0 ± 23.3%) (p < 0.01). For ΔSmO2, no main effect for an interaction was detected, with no main effect of either trial or bout.
Lastly, repeated-measures relative reliability for mean work SmO2 showed good reliability (ICC = 0.87). The SEM for the same variable was 12% (CI 9%–15%).
4. Discussion
The aim of this study was to describe the effect of severe-intensity bouts on SmO2, measured by a wearable NIRS sensor at the VL muscle during self-paced cycling efforts, as well as to examine the reliability of SmO2 between sessions. Our first hypothesis was that SmO2 would desaturate with each bout number. Our results showed an effect of bout on SmO2 within session, but the effect was not as hypothesized. For our second aim, we hypothesized that SmO2 will demonstrate good reliability. No effect of trial was detected across all NIRS outcomes, and no difference was found between sessions, supporting our second hypothesis. Our protocol required each participant to sustain a pre-determined power target, estimated at 5% above their RCP PO, at a repeatable cadence and gear ratio.
From a subgroup analysis (n = 7), no difference in cadence was detected. For the same subgroup, HR response during bout 2 and 4 was significantly elevated relative to bout 1, suggesting a cardiac drift during exercise in the severe intensity domain that agrees with a prior report from Jones et al. (2011).Within each severe-intensity bout, muscle oxygenation displayed an expected desaturation response from the onset to the end of each bout, as seen in previous reports (16, 34–37). Our results showed an increase in onset SmO2 values between the first two bouts and final two bouts, reflecting post-exercise hyperemia, which is a function of metabolic disturbance (38–40). Additionally, work SmO2 significantly increased (i.e., less desaturation) between all bouts, with ΔSmO2 only presenting a significant decrease in amplitude between bouts 3 and 4. Contrary to our second hypothesis, both work SmO2 and ΔSmO2 demonstrated a resaturation response between bouts. Previous studies looking at SmO2 response during both an incremental exercise test, and constant-load exercise, present a continuous desaturation response to the limit of tolerance (14, 27, 37, 41, 42).
With sustained or repeated work bouts in the severe intensity domain, blood flow to working muscles will increase to try to match the demand for O2 as metabolites accumulate (43, 44). The increasing blood flow to the working muscle could be explained by accumulating metabolite concentration within the muscle (45). However, it may be hypothesized that O2 extraction would increase proportionate to O2 delivery, such that muscle V˙O2 would increase, i.e., responsible for the muscular contribution to the V˙O2 slow component during workload within the severe domain (16, 37, 46). Considering the other tissues detected by NIRS, blood flow may be expected to increase to the skin during work for evaporative heat loss. Skin and subcutaneous adipose tissue are less metabolically active, and so an increase in SmO2 would be expected for participants with higher skinfold thickness (9, 10, 47–49). This thermoregulatory cutaneous blood flow would also contribute a larger proportion to the overall NIRS signal (9, 48, 50, 51). As such, given the average skinfold measured at the VL in the current participant group (11.3 ± 5.4 mm), it is possible that the increasing SmO2 response between bouts is not related to changes within muscle, but rather due to non-muscle tissues under the wearable NIRS sensor.
In contrast to our results, Grassi et al. (2003), who investigated the effect of constant workload, high-intensity, cycling bouts on muscle oxygenation in the severe domain, found a continuous desaturation response during the bouts in conjunction with a continuous rise in pulmonary V˙O2 (36). This was further confirmed by Paquette et al. (2020), in trained sprint kayakers across three severe intensity bouts at different distances (200, 500, 1,000 m) using the same NIRS device as our study. We speculate the reason for the discrepancy with our results is due to the lower skinfold thickness reported for the trained kayakers (3.3 ± 0.4 mm) (41).
A study by Kriel, et al. (2018), reported no change in muscle oxygenation during repeated severe-intensity bouts, both in cycling and running exercise modes (23). This disagreement with our results is likely attributable to the difference in work duration spent in the severe domain in each bout. The cumulative time spent in the severe domain in Kriel, et al., was equal to 120 s, compared to 960 s used in this study. It is reasonable to assume that the changes observed in SmO2 response across bouts are related to the total duration or workload performed within the severe domain (16, 36, 37).
Lastly, with regards to test-retest reliability, our results showed that SmO2 measured by a wearable NIRS sensor was able to reliably indicate desaturation response in SmO2 during severe intensity self-paced cycling. Comparisons of the outcome variables between the two sessions showed no effect of trial. Relative and absolute reliability analysis for mean work SmO2 showed good reliability, corresponding to a SEM of ∼12%. Celie et al., reported similar ICC values (ICC = 0.87), when comparing forearm muscle contraction at 70% of maximal voluntary contraction (5). Other studies that measured the reliability of muscle oxygenation during whole-body incremental cycling exercise, reported higher ICC values at the VL (ICC = 0.94) (13). It is worth noting that both studies used a stationary, laser-based NIRS system (OxiplexTS, ISS, Champaign, USA). Also, their exercise protocol included resistance controlled by the ergometer (13). This is different from our self-paced efforts, during which the athlete was asked to match the gearing and cadence to sustain a specific target PO, rather than a fixed resistance set by the ergometer. The ecological nature of our exercise protocol as well as a higher accumulated fatigue, may account for the slightly lower reliability between sessions.
Considering these differences, our results highlight the practical capability of wearable NIRS sensors to provide a reliable test-retest measure of muscle oxygenation response under field-relevant training conditions. It is possible that practitioners can observe a hyperemic response during recovery between bouts, coupled with inhibition in SmO2 desaturation response during severe-intensity bouts, depending on the tissue composition under the wearable NIRS sensor. More work is needed to interpret the effect of tissue composition on this response prior to informing sport practitioners about how to design interval training based on muscle metabolic demand, in conjunction with subjective reporting from the athlete, heart rate data, and external load measures.
5. Limitations
Both HR and cadence were outcome variables of interest. As mentioned previously, measures of PO were measured in all participants, whereas cadence and HR were measured in only seven participants. These variables were not measured for the original sample, however, following a decision to recruit more females for the study, these variables were included as well. Despite the lack of data from the seven males, our sample size (n = 7) presented sufficient power (β = 0.08, α = 0.05) to eliminate a type two error for both HR and cadence (Supplementary Material). Skinfold measurements were obtained, but due to our sample size we were underpowered to analyze between group differences with skinfold as a main effect. Future studies should investigate the effect of body composition on muscle oxygenation from wearable NIRS during severe intensity exercise, as well as other intensity domains.
6. Conclusions
We conclude that SmO2 measurements, using a non-invasive, affordable, wearable NIRS sensor can demonstrate the heterogeneous muscle oxygenation response expected during severe intensity interval exercise on a cycling ergometer with good reliability between sessions in trained cyclists. The within session response presented an effect of bout number on SmO2 desaturation response. Despite hypothesizing SmO2 would desaturate with each bout, it responded in an opposite manner, demonstrating less desaturation with each bout. One explanation may be due to a significant contribution of non-muscular tissue to the O2HbMb signal. Despite finding good, between session reliability, sport practitioners should be aware that further investigation is needed to better understand the effect of severe intensity exercise on muscle oxygen saturation response using wearable NIRS, especially between groups with different body compositions.
Data availability statement
The original contributions presented in the study are included in the article/Supplementary Material, further inquiries can be directed to the corresponding author/s.
Ethics statement
The studies involving human participants were reviewed and approved by University of British Columbia. The patients/participants provided their written informed consent to participate in this study.
Author contributions
AY and JA have equally contributed to the study design, data acquisition, and write up, under the supervision of MK, and guidance of the remaining listed authors. All authors contributed to the article and approved the submitted version.
Funding
This study was funded by a Natural Sciences and Engineering Research Council Discovery Grant and an equipment grant from the British Columbia Sport and Exercise Medicine Research Foundation.
Conflict of interest
The authors declare that the research was conducted in the absence of any commercial or financial relationships that could be construed as a potential conflict of interest.
Publisher's note
All claims expressed in this article are solely those of the authors and do not necessarily represent those of their affiliated organizations, or those of the publisher, the editors and the reviewers. Any product that may be evaluated in this article, or claim that may be made by its manufacturer, is not guaranteed or endorsed by the publisher.
Supplementary material
The Supplementary Material for this article can be found online at https://www.frontiersin.org/articles/10.3389/fspor.2023.1086227/full#supplementary-material.
References
1. Passfield L, Hopker J, Jobson S, Friel D, Zabala M. Knowledge is power: issues of measuring training and performance in cycling. J Sports Sci. (2017) 35(14):1426–34. doi: 10.1080/02640414.2016.1215504
2. Jeukendrup A, VanDiemen A. Heart rate monitoring during training and competition in cyclists. J Sports Sci. (1998) 16:Suppl:S91–9. doi: 10.1080/026404198366722
3. Perrey S, Ferrari M. Muscle oximetry in sports science: a systematic review. Sport Med. (2018) 48(3):597–616. doi: 10.1007/s40279-017-0820-1
4. Tew GA, Ruddock AD, Saxton JM. Skin blood flow differentially affects near-infrared spectroscopy-derived measures of muscle oxygen saturation and blood volume at rest and during dynamic leg exercise. Eur J Appl Physiol. (2010) 110(5):1083–9. doi: 10.1007/s00421-010-1596-2
5. Celie B, Boone J, Van Coster R, Bourgois J. Reliability of near infrared spectroscopy (NIRS) for measuring forearm oxygenation during incremental handgrip exercise. Eur J Appl Physiol. (2012) 112(6):2369–74. Available at: https://link-springer-com.ezproxy.library.ubc.ca/article/10.1007/s00421-011-2183-x. doi: 10.1007/s00421-011-2183-x
6. Calaine Inglis E, Iannetta D, Murias JM. The plateau in the NIRS-derived [HHb] signal near the end of a ramp incremental test does not indicate the upper limit of O2 extraction in the vastus lateralis. Am J Physiol. (2017) 313(6):R723–9. doi: 10.1152/ajpregu.00261.2017
7. Buchheit M, Ufland P, Haydar B, Laursen PB, Ahmaidi S. Reproducibility and sensitivity of muscle reoxygenation and oxygen uptake recovery kinetics following running exercise in the field. Clin Physiol Funct Imaging. (2011) 31(5):337–46. Available at: https://pubmed.ncbi.nlm.nih.gov/21771251/. doi: 10.1111/j.1475-097X.2011.01020.x
8. Perrey S. Muscle oxygenation unlocks the secrets of physiological responses to exercise: time to exploit it in the training monitoring. Front Sports Active Living. (2022) 4:1–4. Available at: https://www.frontiersin.org/article/10.3389/fspor.2022.864825. doi: 10.3389/fspor.2022.864825
9. Barstow TJ. Understanding near infrared spectroscopy and its application to skeletal muscle research. J Appl Physiol. American Physiological Society. (2019) 126:1360–76. doi: 10.1152/japplphysiol.00166.2018
10. McManus CJ, Collison J, Cooper CE. Performance comparison of the MOXY and PortaMon near-infrared spectroscopy muscle oximeters at rest and during exercise. J Biomed Opt. (2018) 23(01):1. doi: 10.1117/1.JBO.23.1.015007
11. Feldmann A, Schmitz R, Erlacher D. Near-infrared spectroscopy-derived muscle oxygen saturation on a 0% to 100% scale: reliability and validity of the Moxy Monitor. J Biomed Opt. (2019) 24(11):1. Available at: https://www.spiedigitallibrary.org/journals/journal-of-biomedical-optics/volume-24/issue-11/115001/Near-infrared-spectroscopy-derived-muscle-oxygen-saturation-on-a-0/10.1117/1.JBO.24.11.115001.full. doi: 10.1117/1.JBO.24.11.115001
12. Spencer MD, Murias JM, Paterson DH. Characterizing the profile of muscle deoxygenation during ramp incremental exercise in young men. Eur J Appl Physiol. (2012) 112(9):3349–60. doi: 10.1007/s00421-012-2323-y
13. Iannetta D, Qahtani A, Maturana FM, Murias JM. The near-infrared spectroscopy-derived deoxygenated haemoglobin breaking-point is a repeatable measure that demarcates exercise intensity domains. J Sci Med Sport. (2017) 20(9):873–7. Available at: http://www.sciencedirect.com/science/article/pii/S1440244017302736. doi: 10.1016/j.jsams.2017.01.237
14. Keir DA, Fontana FY, Robertson TC, Murias JM, Paterson DH, Kowalchuk JM, et al. Exercise intensity thresholds: identifying the boundaries of sustainable performance. Med Sci Sports Exerc. (2015) 47(9):1932–40. doi: 10.1249/MSS.0000000000000613
15. Yogev A, Arnold J, Clarke D, Guenette JA, Sporer BC, Koehle MS. Comparing the respiratory compensation point with muscle oxygen saturation in locomotor and non-locomotor muscles using wearable NIRS spectroscopy during whole-body exercise. Front Physiol. (2022) 13:818733. Switzerland: Frontiers Media S.A. Available at: http://ubc.summon.serialssolutions.com/2.0.0/link/0/eLvHCXMwrV1LT9wwELYquPSCiqCFFlbDDwjEdpLGR55qEY8KivYY-dmuhBzE7krsj-I_MmMvC0KqeuGSgxMr1ow9nrFnvo8xKXbL4o1NaIIV0rbKlqWXvOKODuW9Q_eESyNVeJOqs2D-ohSxjBac5bhnNBeuDlbRTu_L0BqtdBWc5sZQRVEyxrJ8FVslk0xRUsnzrSYGZWo. doi: 10.3389/fphys.2022.818733
16. Kirby BS, Clark DA, Bradley EM, Wilkins BW. The balance of muscle oxygen supply and demand reveals critical metabolic rate and predicts time to exhaustion. J Appl Physiol. (2021) 130(6):1915–27. Available at: https://doi.org/10.1152/japplphysiol.00058.2021. doi: 10.1152/japplphysiol.00058.2021
17. Jones AM, Grassi B, Christensen PM, Krustrup P, Bangsbo J, Poole DC. Slow component of V˙O2 kinetics: mechanistic bases and practical applications. Med Sci Sport Exerc. (2011) 43(11):2046–62. Available at: https://journals.lww.com/acsm-msse/Fulltext/2011/11000/Slow_Component_of_V_O2_Kinetics__Mechanistic_Bases.5.aspx. doi: 10.1249/MSS.0b013e31821fcfc1
18. Poole DC, Schaffartzik W, Knight DR, Derion T, Kennedy B, Guy HJ, et al. Contribution of excising legs to the slow component of oxygen uptake kinetics in humans. J Appl Physiol. (1991) 71(4):1245–60. doi: 10.1152/jappl.1991.71.4.1245
19. McKay AKA, Stellingwerff T, Smith ES, Martin DT, Mujika I, Goosey-Tolfrey VL, et al. Defining training and performance caliber: a participant classification framework. Int J Sports Physiol Perform. (2022) 17(2):317–31. Available at: https://journals.humankinetics.com/view/journals/ijspp/17/2/article-p317.xml. doi: 10.1123/ijspp.2021-0451
20. Van Der Zwaard S, Jaspers RT, Blokland IJ, Achterberg C, Visser JM, Den Uil AR, et al. Oxygenation threshold derived from near- infrared spectroscopy: reliability and its relationship with the first ventilatory threshold. PLoS One. (2016) 11(9):1–16. doi: 10.1371/journal.pone.0162914
21. Crum EM, O’Connor WJ, Van Loo L, Valckx M, Stannard SR. Validity and reliability of the Moxy oxygen monitor during incremental cycling exercise. Eur J Sport Sci. (2017) 17(8):1037–43. doi: 10.1080/17461391.2017.1330899
22. Contreras-Briceño F, Espinosa-Ramirez M, Hevia G, Llambias D, Carrasco M, Cerda F, et al. Reliability of NIRS portable device for measuring intercostal muscles oxygenation during exercise. J Sports Sci. (2019) 37(23):2653–9. Available at: https://pubmed.ncbi.nlm.nih.gov/31419921/. doi: 10.1080/02640414.2019.1653422
23. Kriel Y, Askew CD, Solomon C. The effect of running versus cycling high-intensity intermittent exercise on local tissue oxygenation and perceived enjoyment in 18-30-year-old sedentary men. PeerJ. (2018) 2018(6):1–26. doi: 10.7717/peerj.5026
24. Beaver WL, Wasserman K, Whipp BJ. A new method for detecting anaerobic threshold by gas exchange. J Appl Physiol. (1986) 60(6):2020–7. doi: 10.1152/jappl.1986.60.6.2020
25. Davis JA. Anaerobic threshold: review of the concept and directions for future research. Med Sci Sports Exerc. (1985) 17(1):6–18. PMID: 3884961
26. Iannetta D, Murias JM, Keir DA. A simple method to quantify the V-O 2 mean response time of ramp-incremental exercise. Med Sci Sports Exerc. (2019) 51(5):1080–6. doi: 10.1249/MSS.0000000000001880
27. Murias JM, Keir DA, Spencer MD, Paterson DH. Sex-related differences in muscle deoxygenation during ramp incremental exercise. Respir Physiol Neurobiol. (2013) 189(3):530–6. doi: 10.1016/j.resp.2013.08.011
28. Bourdon PC, Woolford SM, Buckley JD. Effects of varying the step duration on the determination of lactate thresholds in elite rowers. Int J Sports Physiol Perform. (2018) 13(6):687–93. Available at: https://journals.humankinetics.com/view/journals/ijspp/13/6/article-p687.xml. doi: 10.1123/ijspp.2017-0258
29. Jamnick NA, Botella J, Pyne DB, Bishop DJ. Manipulating graded exercise test variables affects the validity of the lactate threshold and V˙O2peak. PLoS One. (2018) 13(7):1–21. doi: 10.1371/journal.pone.0199794
30. Weir JP. Quantifying test-retest reliability using the intraclass correlation coefficient and the sem. J Strength Cond Res. (2005) 19:231–40. doi: 10.1519/15184.1
31. Bartlett JW, Frost C. Reliability, repeatability and reproducibility: analysis of measurement errors in continuous variables. Ultrasound Obs Gynecol. (2008) 31:466–75. Available at: www.interscience.wiley.com. doi: 10.1002/uog.5256
32. Koo TK, Li MY. A guideline of selecting and reporting intraclass correlation coefficients for reliability research. J Chiropr Med. (2016) 15(2):155–63. Available at: https://www.sciencedirect.com/science/article/pii/S1556370716000158. doi: 10.1016/j.jcm.2016.02.012
33. Hopkins WG. Measures of reliability in sports medicine and science. Sport Med. (2000) 30(1):1–15. doi: 10.2165/00007256-200030010-00001
34. Bhambhani YN. Muscle oxygenation trends during dynamic exercise measured by near infrared spectroscopy. Can J Appl Physiol. (2004) 29(4):504–23. Available at: http://www.nrcresearchpress.com/doi/10.1139/h04-033. doi: 10.1139/h04-033
35. Kriel Y, Kerhervé HA, Askew CD, Solomon C. The effect of active versus passive recovery periods during high intensity intermittent exercise on local tissue oxygenation in 18-30 year old sedentary men. PLoS One. (2016) 11(9):1–20. doi: 10.1371/journal.pone.0163733
36. Grassi B, Pogliaghi S, Rampichini S, Quaresima V, Ferrari M, Marconi C, et al. Muscle oxygenation and pulmonary gas exchange kinetics during cycling exercise on-transitions in humans. J Appl Physiol. (2003) 95(1):149–58. Available at: http://www.jap.org. doi: 10.1152/japplphysiol.00695.2002
37. Paquette M, Bieuzen F, Billaut F. The effect of HIIT vs. SIT on muscle oxygenation in trained sprint kayakers. Eur J Appl Physiol. (2021) 121:2743–59. Berlin/Heidelberg: Springer Berlin Heidelberg. Available at: https://go.exlibris.link/c7cgwt74. doi: 10.1007/s00421-021-04743-z
38. Rosenberry R, Munson M, Chung S, Samuel TJ, Patik J, Tucker WJ, et al. Age-related microvascular dysfunction: novel insight from near-infrared spectroscopy. Exp Physiol. (2018) 103(2):190–200. doi: 10.1113/EP086639
39. Soares RN, McLay KM, George MA, Murias JM. Differences in oxidative metabolism modulation induced by ischemia/reperfusion between trained and untrained individuals assessed by NIRS. Physiol Rep. (2017) 5(19):e13384. Available at: http://doi.wiley.com/10.14814/phy2.13384. doi: 10.14814/phy2.13384
40. McLay KM, Gilbertson JE, Pogliaghi S, Paterson DH, Murias JM. Vascular responsiveness measured by tissue oxygen saturation reperfusion slope is sensitive to different occlusion durations and training status. Exp Physiol. (2016) 101(10):1309–18. doi: 10.1113/EP085843
41. Paquette M, Bieuzen F, Billaut F. Effect of a 3-weeks training camp on muscle oxygenation, V˙O2 and performance in elite sprint kayakers. Front Sport Act Living. (2020) 2:1–13. doi: 10.3389/fspor.2020.00047
42. Boone J, Koppo K, Barstow TJ, Bouckaert J. Effect of exercise protocol on deoxy[Hb+Mb]: incremental step versus ramp exercise. Med Sci Sports Exerc. (2010) 42(5):935–42. doi: 10.1249/MSS.0b013e3181c0ecea
43. Calbet JAL, Jensen-Urstad M, Van Hall G, Holmberg H-C, Rosdahl H, Saltin B. Maximal muscular vascular conductances during whole body upright exercise in humans. J Physiol. (2004) 558(1):319–31. doi: 10.1113/jphysiol.2003.059287
44. Calbet JAL, Lundby C, Sander M, Robach P, Saltin B, Boushel R. Effects of ATP-induced leg vasodilation on v˙o2 peak and leg O2 extraction during maximal exercise in humans. Am J Physiol Integr Comp Physiol. (2006) 291(2):R447–53. doi: 10.1152/ajpregu.00746.2005
45. Bailey SJ, Wilkerson DP, DiMenna FJ, Jones AM. EC -Influence of repeated sprint training on pulmonary O2 uptake and muscle deoxygenation kinetics in humans. J Appl Physiol. (2009) 106(6):1875–87. doi: 10.1152/japplphysiol.00144.2009
46. Fukuoka Y, Poole DC, Barstow TJ, Kondo N, Nishiwaki M, Okushima D, et al. Reduction of v˙O2 slow component by priming exercise: novel mechanistic insights from time-resolved near-infrared spectroscopy. Physiol Rep. (2015) 3(6):e12432. Available at: https://physoc.onlinelibrary.wiley.com/doi/abs/10.14814/phy2.12432. doi: 10.14814/phy2.12432
47. Craig JC, Broxterman RM, Wilcox SL, Chen C, Barstow TJ. Effect of adipose tissue thickness, muscle site, and sex on near-infrared spectroscopy derived total-[hemoglobin-myoglobin]. J Appl Physiol. (2017) 123(6):1571–8. doi: 10.1152/japplphysiol.00207.2017
48. Jones S, Chiesa ST, Chaturvedi N, Hughes AD. Recent developments in near-infrared spectroscopy (NIRS) for the assessment of local skeletal muscle microvascular function and capacity to utilise oxygen. Artery Res. (2016) 16:25–33. Available at: https://www.ncbi.nlm.nih.gov/pubmed/27942271. doi: 10.1016/j.artres.2016.09.001
49. Ryan TE, Erickson ML, Brizendine JT, Young HJ, McCully KK. Noninvasive evaluation of skeletal muscle mitochondrial capacity with near-infrared spectroscopy: correcting for blood volume changes. J Appl Physiol. (2012) 113:175–83. doi: 10.1152/japplphysiol.00319.2012
50. Messere A, Roatta S. Influence of cutaneous and muscular circulation on spatially resolved versus standard beer-Lambert near-infrared spectroscopy. Physiol Rep. (2013) 1(7):e00179. Available at: http://doi.wiley.com/10.1002/phy2.179. doi: 10.1002/phy2.179
51. Osawa T, Shiose K, Takahashi H. Tissue blood volume parameters measured by continuous-wave and spatially resolved NIRS show different changes during prolonged cycling exercise. In: Halpern HJ, LaManna JC, Harrison DK, Epel B, editors. Oxygen transport to tissue XXXIX. Cham: Springer International Publishing (2017). p. 249–54. Available at: https://doi.org/10.1007/978-3-319-55231-6_34
Keywords: muscle oxygenation, near-infrared spectroscopy, cycling, severe intensity bouts, wearable, exercise, exercise testing, cardiorespiratory fitness
Citation: Yogev A, Arnold J, Nelson H, Clarke DC, Guenette JA, Sporer BC and Koehle MS (2023) The effect of severe intensity bouts on muscle oxygen saturation responses in trained cyclists. Front. Sports Act. Living 5:1086227. doi: 10.3389/fspor.2023.1086227
Received: 1 November 2022; Accepted: 20 January 2023;
Published: 23 February 2023.
Edited by:
Paul William Macdermid, Massey University, New ZealandReviewed by:
Thomas Jones, Northumbria University, United KingdomJohn McDaniel, Kent State University, United States
© 2023 Yogev, Arnold, Nelson, Clarke, Guenette, Sporer and Koehle. This is an open-access article distributed under the terms of the Creative Commons Attribution License (CC BY). The use, distribution or reproduction in other forums is permitted, provided the original author(s) and the copyright owner(s) are credited and that the original publication in this journal is cited, in accordance with accepted academic practice. No use, distribution or reproduction is permitted which does not comply with these terms.
*Correspondence: Assaf Yogev michael.koehle@ubc.ca Michael S. Koehle yogevass@student.ubc.ca
Specialty Section: This article was submitted to Elite Sports and Performance Enhancement, a section of the journal Frontiers in Sports and Active Living