Reactive Oxygen Species in the Adverse Outcome Pathway Framework: Toward Creation of Harmonized Consensus Key Events
- 1Division of Risk Assessment, Center for Biological Safety and Research, National Institute of Health Sciences, Kawasaki, Japan
- 2Wildlife Toxicology Research Section, Environment and Climate Change Canada, Toronto, ON, Canada
- 3Norwegian Institute for Water Research (NIVA), Oslo, Norway
- 4Norwegian University of Life Sciences (NMBU), Ås, Norway
- 5Centre for Environmental Radioactivity, Norwegian University of Life Sciences (NMBU), Ås, Norway
- 6Korea Institute of Science and Technology (KIST) Europe, Saarbrücken, Germany
- 7Health Canada, Ottawa, ON, Canada
- 8University of Ottawa, Ottawa, ON, Canada
- 9Silent Spring Institute, Newton, MA, United States
- 10Canadian Nuclear Laboratories, Chalk River, ON, Canada
- 11Independent Researcher, Ohrid, North Macedonia
- 12RECETOX, Faculty of Science, Masaryk University, Brno, Czech Republic
- 13U.S. Army Engineer Research and Development Center (ERDC), Vicksburg, MS, United States
- 14Department of Toxicology, University of Würzburg, Würzburg, Germany
- 15National Research Centre for the Working Environment, Copenhagen, Denmark
- 16Organisation for Economic Co-operation and Development (OECD), Paris, France
- 17Group of Alternative Method Development for Environmental Toxicity Testing, IUF—Leibniz-Research Institute for Environmental Medicine, Duesseldorf, Germany
- 18Philip Morris International R&D, Philip Morris Products SA, Neuchatel, Switzerland
- 19Center for Gender-specific Medicine, Italian National Institute of Health, Rome, Italy
- 20University of Helsinki, Ecosystems and Environment Research Programme, Faculty of Biological and Environmental Sciences, Lahti, Finland, and Helsinki Institute of Sustainability Science (HELSUS), Helsinki, Finland
- 21UK Health Security Agency, Public Health England, London, United Kingdom
- 22Universities of Basel and Geneva, Basel, Switzerland
Reactive oxygen species (ROS) and reactive nitrogen species (RNS) are formed as a result of natural cellular processes, intracellular signaling, or as adverse responses associated with diseases or exposure to oxidizing chemical and non-chemical stressors. The action of ROS and RNS, collectively referred to as reactive oxygen and nitrogen species (RONS), has recently become highly relevant in a number of adverse outcome pathways (AOPs) that capture, organize, evaluate and portray causal relationships pertinent to adversity or disease progression. RONS can potentially act as a key event (KE) in the cascade of responses leading to an adverse outcome (AO) within such AOPs, but are also known to modulate responses of events along the AOP continuum without being an AOP event itself. A substantial discussion has therefore been undertaken in a series of workshops named “Mystery or ROS” to elucidate the role of RONS in disease and adverse effects associated with exposure to stressors such as nanoparticles, chemical, and ionizing and non-ionizing radiation. This review introduces the background for RONS production, reflects on the direct and indirect effects of RONS, addresses the diversity of terminology used in different fields of research, and provides guidance for developing a harmonized approach for defining a common event terminology within the AOP developer community.
1 Introduction
Exposure to various types of stressors (e.g., allergens, ionizing radiation, chemicals) can induce cellular-level oxidative damage to macromolecules such as DNA, proteins, and lipids. This is a result of oxidative stress from an imbalance in the production of reactive oxygen species (ROS) in a wide sense. One of the difficulties in the field of ROS research is the use of terminology whose meaning is interpreted differently in the different areas of expertise. To overcome the problems, the Mystery of ROS, an international consortium for creating harmonized Key Events (KEs) on ROS in the Adverse Outcome Pathway (AOP) framework has been established (Tanabe et al., 2022). This review aims to provide some guidance on the definition of the harmonized KEs on ROS in the AOP framework.
The field of ROS in a broad sense has previously been reviewed by Sies et al. (Sies et al., 2017). Non-radical species function as second messengers to regulate life (Sies et al., 2017). Two main types of radicals can be produced including ROS in a narrow sense and reactive nitrogen species (RNS), which are regulated by antioxidant defense response (ADR) that might be defined as radical scavenging mechanisms and oxidant reduction. The ADR constitutes the totality of the activation of processes that protect the cells against ROS. These radicals can be long- and short-lived, depending on their reactivity with other molecules. In order to handle ROS (in a narrow sense) and RNS formation, antioxidant defense mechanisms are recruited to manage the damage. These processes can be classified as enzymatic [e.g., glutathione-S-transferase (GST), catalase (CAT), glutathione-peroxidase (GPX), and superoxide dismutase (SOD)] and non-enzymatic systems (e.g., uric acid, vitamin C and E and lipoic acid). Normally, these are the first line of defense and these can act as oxidant scavengers. Oxidative stress is defined as a condition where the ROS production is sustainably excessive beyond ADRs and plays a central role in pathological processes including cancer, diabetes, chronic kidney disease, neurodegenerative disease, cardiovascular disease, chronic obstructive pulmonary disease (Dröge, 2002; Liguori et al., 2018). ROS are also crucial in generating immune responses, signaling injury, and upregulating inflammatory responses, thereby strongly affecting various disease pathogenesis, including cancer and bacterial and viral infection including severe acute respiratory syndrome coronavirus-2 (SARS-CoV-2) causing the current global pandemic coronavirus infectious disease (COVID-19) (Liou and Storz, 2010; Cecchini and Cecchini, 2020; Saleh et al., 2020; Shenoy, 2020; Alam and Czajkowsky, 2021; Kalyanaraman, 2021). ROS and oxidative stress have been implicated as potential key contributors to toxic effects mediated by pollutants, radiation, and nanoparticles. Environmental stressors such as pollutants and radiation often contribute to the exaggeration of oxidative stress (Table 1).
2 Intracellular Sources of ROS and Initiating Events
ROS can be both KEs in the AOP leading to the Adverse Outcome (AO) or associated where their formation is a consequence of the AO rather than a cause of that AO. For all environmental stressors, it is crucial to make this distinction. This can be achieved by determining the temporal relationship of ROS formation relative to the AO or examining the Molecular Initiating Event (MIE). Where for example, the MIE is likely to generate ROS such as a redox cycling or effect on complex I there is a higher probability of ROS being a KE in the AOP than where the MIE is something unrelated to ROS such as direct reaction with protein or DNA.
2.1 Direct Sources of ROS
Radiation can increase immediate and longer-term ROS. Comprehensive mechanisms of ROS-dependent and independent DNA damage induced by ionizing radiation and solar radiation have been proposed (Cadet and Wagner, 2013; Helm and Rudel, 2020). Radiation can also increase antioxidants such as SOD, CAT, and peroxidase (POD) (Seen and Tong, 2018; Ahmad et al., 2021). Radiation-induced DNA damage and ROS can also lead to cell death and autophagy and are utilized for this reason in cancer treatment (Ma et al., 2021; Yamamoto et al., 2021). The differences between high and low linear energy transfer (LET) should be considered in terms of ROS production and DNA damage in the radiation fields of cancer since high LET deposits dense clusters of energy closer to the tissue surface and creates more complex DNA damage (Goodhead, 1988).
2.2 Indirect Sources of ROS
ROS are commonly involved in the mode of action (MoA) of various classes of environmental chemicals including metals such as lead, chromium, arsenic, mercury, nickel, and cadmium (Renu et al., 2021), pesticides such as pyrethroids, carbamates and organophosphates (Medithi et al., 2021), and other industrial chemicals such as bisphenol A (Steffensen et al., 2020). ROS production contributes to a large variety of environmentally-induced diseases (Omidifar et al., 2021), e.g., oxidative stress is one of the most important mechanisms of action of pesticides in acute and chronic poisoning (Lukaszewicz-Hussain, 2010), and of metals in hepatotoxicity (Renu et al., 2021). Mitochondria are one of the main producers of ROS in eukaryotic cells. The sources of ROS include mitochondria in muscle cells, nicotinamide adenine dinucleotide phosphate (NADPH) oxidase (NOX), phospholipase A2 (PLA2), xanthine oxidase, monoamine oxidase (MAO), dehydrogenase, and immune cells such as macrophages, eosinophils, monocytes or neutrophils (Powers and Jackson, 2008; Vargas-Mendoza et al., 2021). Free adenosine diphosphate (ADP), inorganic phosphate (Pi), and O2 activate the electron transport chain (ETC) in mitochondria (Hargreaves and Spriet, 2020; Vargas-Mendoza et al., 2021). Other sources are also important as ROS producers. Angiotensin II (Ang II), the main product and effector of the renin-angiotensin system stimulation, is a regulator of blood pressure and produces ROS by stimulating NOX (Nguyen Dinh Cat et al., 2013; Forrester et al., 2018; Pothen and Balligand, 2021). Ang II-induced ROS production leads to mitogen-activated protein kinase (MAPK) activation, where ROS play a role as a second messenger (Nishida et al., 2005; Forrester et al., 2018). When excess Ang II binds to the Ang II Type 1 Receptor (AT1R), several signaling pathway cascades, including the ROS signaling. The formation of ROS through NOX activation further regulates AT1R through a feed-forward mechanism, which amplifies the ROS production with a feed-forward mechanism (Datla and Griendling, 2010; Frazziano et al., 2014). There are varying NOX isoforms that could possibly be engaged in this mechanism which includes NOX 4 isoform located in mitochondria (Veith et al., 2019; Fukai and Ushio-Fukai, 2020). The NOX-derived ROS stimulates peptides and cell-surface receptors to initiate intracellular signaling pathways. Both the ROS formulation and Ang II/AT1R stimulations regulate MAPK pathways, modulating the transcription factors. Multiple MAPK-mediated transcription factors drive the accumulation of myofibroblasts and the development of lung fibrosis (Zhao et al., 2007; Hardie et al., 2009). In the case of alveolar cells, Ang II accumulates at the cellular level resulting in inflammation and lung fibrosis as an AO (Parimon et al., 2020; Shi et al., 2021). Still, the non-converted Ang II, induced by angiotensin converting enzyme (ACE) II downregulation, could circulate to other parts of the body, such as the live heart, skin, kidneys, blood vessels, skeletal muscles, and brain, causing similar AT1R activation results in other organs to induces ROS mediated vasoconstriction, proliferation, inflammation, and fibrosis. In addition, some nanomaterials, especially carbon-based nanomaterials, are efficient ROS producers (Jacobsen et al., 2008).
2.3 Methodologies for Measuring Oxidative Stress
ROS can be detected by intracellular ROS assay and in vitro ROS/RNS assay. Nitric oxide can be detected in intracellular nitric oxide assay. ROS detection in biological systems includes spectrophotometry methods, fluorescence and chemiluminescence methods, and electron-spin resonance (ESR), which requires probes that produce stable products (Dikalov and Harrison, 2014; Zhang et al., 2018). Adducts produced by covalent binding with free radicals can be detected by ESR (Dikalov and Harrison, 2014). Hydroxyl, peroxyl, or other ROS can be measured using a fluorescence probe, 2′, 7′-dichlorodihydrofluorescein diacetate (DCFH-DA), at fluorescence detection at 480 nm/530 nm. Recent progress in ROS measurement includes the development of a standard operating procedure (SOP) for DCFH-DA acellular assay (Boyles et al., 2022). Chemiluminescence analysis can detect the superoxide, where some probes have a wider range for detecting hydroxyl radical, hydrogen peroxide (H2O2), and peroxynitrite (Fuloria et al., 2021). ROS in the blood can be detected using superparamagnetic iron oxide nanoparticles (SPION)-based biosensor (Lee et al., 2020). H2O2 can be detected with a colorimetric probe, which reacts with H2O2 in a 1:1 stoichiometry to produce a bright pink colored product, followed by the detection with a standard colorimetric microplate reader with a filter in the 540–570 nm range. The levels of ROS can be quantified using multiple-step amperometry using a stainless steel counter electrode and non-leak Ag|AgCl reference node (Flaherty et al., 2017). Singlet oxygen can be measured by monitoring the bleaching of p-nitrosodimethylaniline at 440 nm using a spectrophotometer with imidazole as a selective acceptor of singlet oxygen (Onoue et al., 2013).
The level of CAT, GPX, or SOD can be measured as enzymes in the cellular oxidative defense system. CAT is an anti-oxidative enzyme that catalyzes the resolution of H2O2 into H2O and O2. The chemiluminescence or fluorescence of HRP catalytic reaction can be detected with residual H2O2 and probes [DHBS + AAP, or ADHP (10-acetyl-3, 7-dihydroxyphenoxazine)]. Anti-oxidant capacity is also one of the oxidative stress markers. Oxygen radical antioxidant capacity, hydroxyl radical antioxidant capacity, total antioxidant capacity, the cell-based exogenous antioxidant assay can be used for measuring the antioxidant capacity. Oxidation of protein can be measured by the detection of protein carbonyl content (PCC), 3-nitrotyrosine, advanced oxidation protein products, or BPDE protein adduct. DNA oxidation can be detected with 8-oxo-dG/8-hydroxy-2′-deoxyguanosine (8-OHdG) by ELISA. Lipid peroxides decompose to form malondialdehyde (MDA) and 4, hydroxynonenal (4-HNE), natural bi-products of lipid peroxidation. Lipid peroxidation can be monitored by thiobarbituric acid (TBA) reactive substances in biological samples. MDA and TBA form MDA-TBA adduct in a 1:2 stoichiometry and are detected by colorimetric or fluorometric measurement. While direct ROS determination, a promising oxidative stress biomarker, is challenging due to the short half-life and high reactivity of ROS, detection of the resulting oxidative damage to biomolecules (DNA, lipids, and proteins) and antioxidant status (enzymatic antioxidant activities and non-enzymatic antioxidant levels) is more reliable in biological setting (Katerji et al., 2019).
3 Relationships Between ROS and Pathogenesis
3.1 The Roles of ROS in Diseases
Prolonged ROS and oxidative stress mediate a variety of diseases (e.g., cancer, neurological disorders, cardiac diseases, pulmonary diseases), indicating that ROS and oxidative stress can be causal factors as well as biomarkers of diseases (Annesley and Fisher, 2019; Kay et al., 2019; Climent et al., 2020; Ghezzi, 2020). ROS can be classified as free radicals (Table 2) and non-radical (Table 3) ROS. The term RNS refers to both nitrogen-centered radicals and other reactive molecules, which can induce nitrosative stress (e.g., nitric oxide, nitrogen dioxide, nitrous acid, peroxynitrite, dinitrogen trioxide) (Powers and Jackson, 2008). In general, the timely detection of “live-ROS” is difficult since ROS have an extremely short half-life (t½ in seconds). On the other hand, the level of antioxidant enzymes and products of oxidation can be detected as indicators of redox state and oxidative stress, respectively (Gornicka et al., 2011). For instance, 7, 8-dihydro-8-oxo-2′-deoxyguanosine or 7, 8-dihydro-8-oxoguanosine can be detected as a DNA damage biomarker in cancer, and advanced glycation end products or MDA are biomarkers in diabetes (Liguori et al., 2018). An antioxidant enzyme NAD(P)H quinone dehydrogenase 1 (NQO1) is up-regulated while CAT is down-regulated in nonalcoholic steatohepatitis livers (Gornicka et al., 2011).
3.1.1 ROS and DNA Damage
ROS cause a variety of DNA lesions and the resulting cellular responses involve complex crosstalk between multiple repairs and signaling pathways (Kay et al., 2019). ROS induce DNA damage such as the formation of 8-oxoguanine (Kay et al., 2019; Schaich and Van Houten, 2021), the most abundant oxidative DNA lesion, and other types of nucleotide oxidation, deamination, and lipid peroxidation-derived adducts. DNA repair pathways for oxidative DNA lesions include base excision repair and to a lesser extent, nucleotide excision repair (Freudenthal et al., 2017). Failure to repair oxidative lesions prior to replication can lead to mutations (Sasaki et al., 2020). Alternatively, ROS can produce single-strand DNA breaks directly or indirectly (through repair intermediates), replication stress, and the formation of double-strand breaks (DSBs) (Kay et al., 2019). DSB repair occurs by the error-prone non-homologous end-joining pathway or by homologous recombination repair (Kay et al., 2019). Upon the occurrence of DSBs induced by ROS, histone H2AX nearby DSBs is phosphorylated, and phosphorylated H2AX (γ-H2AX) induces DNA damage responses (Ishida et al., 2014). Polyphenols and flavonoids protect DNA from ROS-induced oxidative damage (Khalil Alyahya et al., 2021). DNA damage response networks including pathways leading to the formation of mutations and chromosomal aberrations are involved in cancer (Tanabe et al., 2021).
3.1.2 ROS and Cancer
Cancer is the first or second leading cause of death before the age of 70 years in 112 of 183 countries, according to estimates from the World Health Organization (WHO) in 2019 (Organization, 2020; Sung et al., 2021). While ROS can be a cause of cancer, they can also have a protective effect: e.g., promotion of oxidative stress-induced cancer cell death caused by excessive ROS-induced oxidative damage, and tumor formation in redox-dependent and pro-oncogenic signaling pathways (De Logu et al., 2021; He et al., 2021). Many mechanisms are conserved in different types of cancer and have similar carcinogenic effects (Kay et al., 2019). ROS-induced DNA damage promotes inflammation and cancer (Helm and Rudel, 2020). Epithelial-mesenchymal transition (EMT), a transition of cells from epithelial to mesenchymal state, is the main hallmark of cancer malignancy (Hanahan and Weinberg, 2011; Tanabe et al., 2020; Tanabe et al., 2021). ROS production is involved in the EMT process in cancer (Kozak et al., 2020). Sustained chronic ROS is linked to the poor prognosis of cancer. The expression of NOX4, one of the main sources of ROS, is correlated with tumor size, lymphatic metastasis, and vascular invasion, and thus poor prognosis in gastric cancer (Du et al., 2019). Conversion of fibroblast growth factor receptor 2b (FGFR2b) to FGFR2c induces EMT and represses nuclear factor-erythroid 2 (NFE2) like basic leucine zipper (bZIP) transcription factor 2 (NRF2)-mediated detoxification of ROS, which leads to the progression of cancer (Katoh and Katoh, 2009). ROS mediate the transformation of the tumor microenvironment in radiotherapy-resistant gastric cancer (Gu et al., 2018). Chloroquine-induced ROS production inhibits autophagy and promotes EMT and malignancy in estrogen receptor-positive breast cancer cells (Rojas-Sanchez et al., 2021). Since autophagy inhibition could be a potential cancer treatment, cancer subtypes need to be considered in the application of therapeutics (Rojas-Sanchez et al., 2021). The evidence supports the involvement of ROS in treatment-resistant cancer. Some controversy regarding the role of ROS in cancer progression is ongoing; for example, heat shock protein 27 (HSP27), a stress-induced molecular chaperone, inhibits ROS accumulation, while its expression is associated with metastasis and poor prognosis in cancer (Nagata et al., 2013).
3.1.3 ROS and Infection
Similar to the double role in cancer, the roles of ROS in infection represent the “double-edged sword” concept. The relationships between oxidative stress responses and coagulation in terms of SARS-CoV-2 infection are yet to be determined (Cecchini and Cecchini, 2020). Mesenchymal stem cells (MSCs) are one of the candidate therapies for COVID-19 to reduce inflammation and promote lung regeneration in severe COVID-19 patients (Rocha et al., 2020). MSCs express SOD, which converts superoxide anion to H2O2 and free oxygen, preventing the destruction of surrounding tissue by ROS from neutrophils and M1 macrophages (Jiang et al., 2016; Rocha et al., 2020). AOP379 regarding coronavirus infection and ROS has been developed as an OECD project (https://aopwiki.org/aops/379), in collaboration with an international consortium, the Modelling the Pathogenesis of COVID-19 Using the AOP Framework (CIAO) (Clerbaux, 2022). It is expected that research in this field will develop in the future, including involvement in the immune response of RNA signal networks.
3.2 ROS and Nanoparticles (NPs)
The AOPs leading to effects on the liver by titanium dioxide (TiO2) have been developed, where ROS generation is a KE in the AOP network (Brand et al., 2020). Exposure to TiO2 seems to trigger ROS generation and oxidative stress (Brand et al., 2020). The estimated cumulative dose of 102–104 mg/kg bw, or less than 103 mg/kg bw of TiO2 induces ROS generation, or oxidative stress, respectively. In contrast, a wide range of the cumulative concentration (102–1012 mg/kg bw) of TiO2 induces preneoplastic lesions (Braakhuis et al., 2021). Prolonged inhalation of TiO2 causes its deposition in the lung, which leads to ROS generation (considered as a KE) and oxidative stress (considered as another KE) in lung adenomas/carcinomas (Braakhuis et al., 2021). While nanomaterials potentially induce ROS (Jacobsen et al., 2008) and oxidative stress (Halappanavar et al., 2021), nanoparticle (NP)-based ROS-scavenging approaches have also emerged as nanomedicines for anti-inflammatory treatments (Huang et al., 2021). These ROS-scavenging NPs include catalytic NPs that have SOD-, CAT-, POD-, and glutathione-like enzyme activities, free-radical trapper NPs such as fullerene that captures ROS via conjugated double bonds, 2, 2, 6, 6-tetramethylpiperidinenoxyl (TEMPO) that captures ROS via the single electron on nitroxide, and redox ROS-scavenging NPs such as curcumin or bilirubin NPs (Nash and Ahmed, 2015; Huang et al., 2021). Specific ROS-targeting NPs as drug delivery systems, where solubility changes by ROS or extended-release are utilized for anti-cancer therapy, have been developed as well (Kim et al., 2015). Conversely, carbon black NPs have been shown to induce ROS, oxidative DNA damage, and a ROS-specific mutation spectrum (Jacobsen et al., 2007; Jacobsen et al., 2008; Jacobsen et al., 2011; Modrzynska et al., 2018). In addition, a SOP for assessment of ROS generation using DCFH-DA acellular assay was developed (Boyles et al., 2022). Future studies with stable detection of ROS induced by nanoparticles would reveal the controversial effects of NPs in terms of the relationship between ROS and pathological changes.
3.3 ROS and Radiation Toxicity
Low level radiation is omnipresent due to exposure to natural radionuclides, cosmic and solar radiation and widespread use in various industrial applications (Zdrojewicz et al., 2016). It comes in different forms including both ionizing (e.g., alpha particles, heavy ions, neutrons, beta electrons, gamma photons, X-rays and UVC) and non-ionizing radiation (e.g., UVB, UVA, visible light, microwave, radio and other low-frequency radiation). Ionizing radiation arises from many sources including natural (e.g., Naturally Occurring Radioactive Materials (NORM) and cosmic rays), medical (e.g., radiotherapy), diagnostic (e.g., computed tomography) and anthropogenic activities such as mining and milling (e.g., Technologically Enhanced Naturally Occurring Radioactive Material (TENORM)). Severe adverse effects of ionizing radiation has predominantly been associated with acute exposures to high radiation dose rates from nuclear accidents and nuclear detonations, albeit prolonged exposure to low radiation dose rate is considered relevant for a number of chronic pathologies. Non-ionizing radiation such as solar UV (UVA and UVB) and anthropogenic use of high-energy UVC radiation in disinfection etc. are potential sources for both acute and chronic effects. Although UV radiation is considered highly relevant for human and environmental health, ionizing radiation are often highlighted for its high ROS-inducing potential.
When ionizing radiation interacts with the high-water content of cells, the ionization events generate a variety of molecular species from water radiolysis which include the hydrated electron, radicals such as hydroxyl, hydride, superoxide, and molecular species such as H2O2 and di-hydrogen (Gebicki, 2016). The production of ROS from these interactions is therefore secondary to the initial physical interaction with biological matter (Alizadeh et al., 2015). Once formed, these species can also induce damage, alter the redox environment in proximity of the track and/or mimic signaling pathways that involve ROS produced by other mechanisms such as mitochondrial sources (Breitenbach and Eckl, 2015; Sies, 2015; Tharmalingam et al., 2017). The extent of ionization events and follow-on ROS-induced toxicity pathway activation can be influenced by the particle size and initial kinetic energy which is often a function of dose of exposure and dose-rate of delivery. In the case of photons that have no mass, the incident particle is an electron with an energy related to the incident photon wavelength. The physiological consequences are wide-ranging depending on the extent and specific site of damage. Studies have shown oxidative stress from radiation exposure can lead to neurotoxicity closely associated with Alzheimer’s disease, developmental disorders (Cheignon et al., 2018), reproductive disruption (Xie et al., 2019; Song et al., 2020), growth inhibition (Xie et al., 2019) and cardiovascular diseases (Ahotupa, 2017). However, studies have also shown that ROS production is not necessarily detrimental and can have an adaptive role, particularly at low levels, enhancing the resistance of cells to oxidative damage from higher levels of radiation exposure (Buonanno et al., 2011). Additionally, co-exposure to radiation with other environmental stressors and cellular toxins often causes augmented biological effects mediated through ROS production (Salbu et al., 2019). Additionally, factors such as endogenous production of ROS, nutritional and antioxidant status, age, life stage differences and individual as well as epigenetic variations are known modulators of ROS formation and further influence radiation-induced toxicity.
3.4 Biomarkers of ROS
Biomarkers have been defined by the U.S. Food and Drug Administration and the National Institutes of Health as measurable characteristics, which are evaluated as indicators of a biological process, pathogenic process, or pharmacological response (Califf, 2018). Biomarkers of effect are crucial for determining the formation of ROS in the AOP and their role in the AOP as they can often serve as a readout for KEs or associated events in the causal chain of events leading to an AO. Macromolecular degradation products might serve as biomarkers of the effect of ROS in a causal chain of events leading to the AO such as cellular/organ structural degeneration. In the case of gene expression alterations, it may be more suitable to call the event an associated event rather than a KE, since the genes induced may encode cytoprotective proteins that seek to mitigate it. Thereby differences in organ-specific antioxidative defenses and basic ROS levels need to be considered (Scandalios, 2005). Several biomarkers of ROS can be assessed in dependence on in vitro or in vivo contexts, and at different levels of biological organization (Ho et al., 2013). The products of in vivo ROS can be measured in body fluids such as blood or urine. Examples of such are biomarkers concerning lipid peroxidation like MDA, protein damage like 3-nitro-tyrosine (3-NO2Tyr), DNA/RNA damage like 8-OHdG or 8-nitro-guanine (8-NO2Gua) or general biomarkers such as glutathione (oxidized/reduced ratio) (Porter et al., 1995; Meagher and FitzGerald, 2000; Smith et al., 2011; Steffensen et al., 2020). F2-isoprostanes, prostaglandin-like compounds formed by non-enzymatic free radical-induced peroxidation of arachidonic acid, can be biomarkers for monitoring ROS and oxidative stress (Milne et al., 2011; Ma et al., 2017). In addition, in vitro ROS can directly be measured by using fluorescent dyes. Here, fluorescent probes such as DCFH-DA detected cytosolic ROS (Xie et al., 2019; Song et al., 2020), while dihydrorhodamine 123 (DHR123) and C11-BODIPY were applied for the detection of mitochondrial and lipid peroxidation-related ROS, respectively (Gomes et al., 2017; Song et al., 2020). Also, changes in antioxidative gene expression can serve as the first indication of exposure exceeding basal defense levels. Differential expression of genes coding for antioxidative enzymes such as SOD1, heme oxygenase 1 (HMOX1), oxidative stress-induced growth inhibitor 1 (OSGIN1), NQO1 or glutamate-cysteine ligase modifier (GCLM) can be indicative, although there is no established signature of differential gene expression profile for ROS (Yang and Chitambar, 2008; Gornicka et al., 2011; Yi et al., 2014; Tsai et al., 2017). There is not a consistent understanding of how changes in expression of genes indicate ROS levels. For instance, NQO1 is up-regulated while CAT is down-regulated in nonalcoholic steatohepatitis livers (Gornicka et al., 2011). HMOX1 is up-regulated and CAT is down-regulated by gallium nitrate in human lymphoma cells (Yang and Chitambar, 2008). Moreover, levels of antioxidant enzymes [SOD, CAT, POD, GST, GPX or glutathione-reductase (GR)] can be determined as indicators of redox state and oxidative stress (Scandalios, 2005). Especially, gene expression changes might be used as a first-line screening for a MoA of in vitro ROS. This was recently exemplified in a study examining the MoA of herbal medicines. Here, ROS-dependent induction of antioxidative gene expression serves as an event in the generated putative AOP “Disturbance of oligodendrocyte differentiation/maturation leading to intellectual disability due to alterations in white matter” (Klose et al., 2022). For the determination of the role of ROS as a KE within an AOP, the most sensitive biomarkers of ROS need to be utilized. Either a direct measurement of ROS or the use of some of the most sensitive gene expression biomarkers such as HMOX1 and NQO1 would appear to be the optimal methods. These must be measured in a time-based manner relative to the AO to carefully distinguish whether the ROS formation is a key or associated event in relation to the AO. Furthermore, care must be taken with the biological system, and in particular in vitro where the high partial pressure of oxygen can result in the formation of ROS which may not be relevant in the low oxygen partial pressure environment of the organ (Ortiz-Prado et al., 2019).
4 Creation of Harmonized ROS-Related KEs
The Mystery of ROS consortium aims to create harmonized ROS-related KEs. One umbrella event capturing all components such as “imbalance of oxidative stress processes” could be considered. Alternatively, ROS and RNS could be combined to “reactive oxygen and nitrogen species (RONS)” and ADR represented separately, as the role of ADR in disease/adversity progression is well-defined. There is an obvious challenge of providing sufficient specificity in the KE description to balance producing a general KE that can also be reused in the AOP framework as a modular unit in different related pathways. Similarly, another challenge is identifying which measurement methods to include in the description such that the KE remains broad and not overly specific. Directionality of KEs defined as either “up” or “down” would also need consideration. It has been considered whether the terminology of “up-regulation of ROS” and “increase in ROS” is different or not, since “up-regulation of ROS” rather means active production of ROS in cellular mechanism, while “increase in ROS” captures both aspects of the excessive production of ROS and decrease in ADR. Active regulation of ROS, which the term “up-regulation of ROS” may imply, may fit better to certain cellular or molecular reactions in disease progression (e.g., inflammatory responses, immune responses, etc.), however, may not always fit in the events of ROS produced or formed directly due to the oxidative reactions involving exogenous stressors. Naming such as “excessive RONS production” would be another possibility, however, would need a clearer definition of what “excessive” means and is highly dependent on the ADR status, and therefore challenging to determine without taking into account downstream events of the AOP to define a threshold for what is considered “excessive”. The consortium also discussed “Depletion of protective oxidative stress response,” this term was not favored as it indicates that the protective responses can be exclusively associated with one mechanism of action. The ADR represents both depletion of cellular antioxidants and induction of protective enzymes that regenerate cellular reduction potential (e.g., reducing oxidated proteins) or enzymatically reduce a radical species. The term “depletion” therefore does not cover the diversity of the ADR system, and terminology such as “exhaustion” or similar terminology such as “diminished” may be more appropriate. An alternative naming considered would be “insufficient antioxidant defenses” which is defined in terms of a state or condition that may not be easily determined. However, none of these terms cohere well with defining the directionality for easy integration into the AOP-Wiki (https://aopwiki.org). As both depend on the RONS production (endogenous/exogenous) or the demonstration of the onset/triggering of downstream KEs to indicate a departure from the condition “sufficient”, they may thus not be ideal terms for the complex or totality of the ADR. A general term such as “increase, (protective) oxidative defense response” (directional) or “altered (protective) oxidative defense response” (undirectional) would capture the naming of events crucial for the AOP itself (i.e., a KE) as well as modifying factors that would not necessarily be considered as a KE in the AOP. The main objectives of KE harmonization described in this manuscript were to estimate existing knowledge of general ROS regarding the AOP framework and its application and gather ideas and input from the broader scientific community that can help guide and direct further development of the AOP framework. These initiatives of the workshop have provoked a discussion among research scientists in moving the Science of AOPs forward to consider how best to use and optimize the ROS-driven oxidative stress in the regulatory AOP framework. Therefore, it is relevant to hazard and risk assessment that research and regulatory communities identify current limitations of undefined ROS KEs and their qualitative and quantitative implications to guide data curation to ensure consistency for oxidative stress-specific AOPs and their applications. Identification of the therapeutic targets or prediction of adverse effects of therapeutics in diseases utilizing the AOP framework would be one of the future directions (Figure 1).
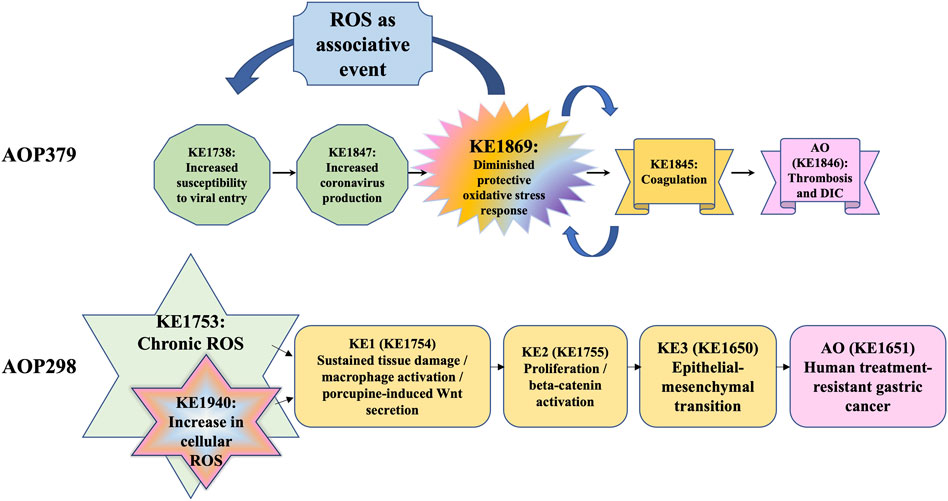
FIGURE 1. Examples for the concept of the ROS as a KE and associative event in AOPs *DIC: disseminated intravascular coagulation.
5 Conclusion
ROS produced in response to stimuli play various roles and have many faces in human health and diseases. The beneficial roles and adverse effects of ROS should be considered to solve the puzzles of AOP constructs with existing insights in the ROS field. The mystery of ROS consortium continues the effort to harmonize the events in ROS-related networks. The aim of this review was to clearly show where ROS are likely to be a KE within an AOP, and the role of the ADR to mitigate the effect of ROS and thus modulate the magnitude of the associated AO. There is a clear need to differentiate in an AOP where ROS are a KE and where they are associated events. This can be done by understanding the temporal sequence of events associated with ROS and where they occur relative to the AO and the KEs likely associated with the MIE (Figure 1). Further discussion would continue in the Mystery of ROS consortium in the future.
Author Contributions
ST drafted, reviewed and edited a manuscript. EF and JöK drafted section 3.4 Biomarkers of ROS. KT, VC, and DB drafted 3.3 ROS and radiation toxicity, and reviewed and edited the manuscript. CY and EH drafted 3.1.1 ROS and DNA damage, and reviewed and edited the manuscript. RR, JeK, and JH drafted 3.1.1 ROS and DNA damage, 3.1.2 ROS and cancer, and reviewed and edited the manuscript. ME drafted 1 Introduction, and reviewed and edited the manuscript. TG reviewed and edited the abstract and manuscript. YK, JF, IS, and RF reviewed and edited the manuscript. JO’B, NG-R, AM, SP, ND, KL, CLR, HY, PD, NJ, UV, and IC reviewed and edited the manuscript. All sections including 1 Introduction, 2 Intracellular sources of ROS and initiating events, 3 Relationships between ROS and pathogenesis, 4 Creation of harmonized ROS-related KEs and 5 Conclusion are collaborative works of the authors. All authors read, commented, and approved for the publication.
Funding
This research was supported by Japan Agency for Medical Research and Development (AMED) Grant Numbers JP21mk0101216 (ST) and JP22mk0101216 (ST), JSPS KAKENHI Grant Number 21K12133 (ST), Research Council of Norway (RCN) grants 223268 and 160016 (KT), NIVAs Computational Toxicology Program, NCTP (www.niva.no/nctp) (KT), the European Union’s Horizon 2020 research and innovation programme under Grant Agreement No. 857560 (IS), and the National Research Council of Science & Technology (NST) Grant by the Strategies for Establishing Global Research Networks (No. Global-21-00X) (YK, IC). This work was supported by the Ministry of Health, Labour, and Welfare (MHLW), Japan.
Conflict of Interest
KL and HY were employed by the company Philip Morris International (PMI).
The remaining authors declare that the research was conducted in the absence of any commercial or financial relationships that could be construed as a potential conflict of interest.
Publisher’s Note
All claims expressed in this article are solely those of the authors and do not necessarily represent those of their affiliated organizations, or those of the publisher, the editors and the reviewers. Any product that may be evaluated in this article, or claim that may be made by its manufacturer, is not guaranteed or endorsed by the publisher.
Acknowledgments
The authors would like to acknowledge Sabina Halappanavar, Dan Villeneuve, Prof. Pietro Ghezzi, Ed Perkins, Penny Nymark, Magdalini Sachana, Brigitte Landesmann, Carlie LaLone, Kristie Sullivan, Tanja Burgdorf, Michelle Angrish, Bette Meek, and Maria João Amorim, all participants in the Mystery of ROS consortium, OECD AOP Coach Team members and EAGMST members. The authors would like to thank members of the National Institute of Health Sciences (NIHS), Japan. Any opinions, findings, and conclusion or recommendations expressed in this material are those of the authors and do not necessarily reflect the views of the authors’ organization, the European Commission in European Union (EU), JSPS, Ministry of Education, Culture, Sports, Science and Technology-Japan (MEXT), or MHLW in Japan.
References
Ahmad, S., Hussain, A., Ullah, F., Jamil, M., Ali, A., Ali, S., et al. (2021). 60Co-γ Radiation Alters Developmental Stages of Zeugodacus Cucurbitae (Diptera: Tephritidae) through Apoptosis Pathways Gene Expression. J. Insect Sci. 21 (5), 16. doi:10.1093/jisesa/ieab080
Ahotupa, M. (2017). Oxidized Lipoprotein Lipids and Atherosclerosis. Free Radic. Res. 51 (4), 439–447. doi:10.1080/10715762.2017.1319944
Aid, M., Busman-Sahay, K., Vidal, S. J., Maliga, Z., Bondoc, S., Starke, C., et al. (2020). Vascular Disease and Thrombosis in SARS-CoV-2-Infected Rhesus Macaques. Cell. 183 (5), 1354–1366. e1313. doi:10.1016/j.cell.2020.10.005
Alam, M. S., and Czajkowsky, D. M. (2022). SARS-CoV-2 Infection and Oxidative Stress: Pathophysiological Insight into Thrombosis and Therapeutic Opportunities. Cytokine & Growth Factor Rev. 63, 44–57. doi:10.1016/j.cytogfr.2021.11.001
Alizadeh, E., Orlando, T. M., and Sanche, L. (2015). Biomolecular Damage Induced by Ionizing Radiation: The Direct and Indirect Effects of Low-Energy Electrons on DNA. Annu. Rev. Phys. Chem. 66 (1), 379–398. doi:10.1146/annurev-physchem-040513-103605
Annesley, S. J., and Fisher, P. R. (2019). Mitochondria in Health and Disease. Cells 8 (7), 680. doi:10.3390/cells8070680
Bhattacharyya, A., Chattopadhyay, R., Mitra, S., and Crowe, S. E. (2014). Oxidative Stress: an Essential Factor in the Pathogenesis of Gastrointestinal Mucosal Diseases. Physiol. Rev. 94 (2), 329–354. doi:10.1152/physrev.00040.2012
Boyles, M., Murphy, F., Mueller, W., Wohlleben, W., Jacobsen, N. R., Braakhuis, H., et al. (2022). Development of a Standard Operating Procedure for the DCFH2-DA Acellular Assessment of Reactive Oxygen Species Produced by Nanomaterials. Toxicol. Mech. Methods 32, 439–452. doi:10.1080/15376516.2022.2029656
Braakhuis, H. M., Gosens, I., Heringa, M. B., Oomen, A. G., Vandebriel, R. J., Groenewold, M., et al. (2021). Mechanism of Action of TiO2: Recommendations to Reduce Uncertainties Related to Carcinogenic Potential. Annu. Rev. Pharmacol. Toxicol. 61 (1), 203–223. doi:10.1146/annurev-pharmtox-101419-100049
Brand, W., Peters, R. J. B., Braakhuis, H. M., Maślankiewicz, L., and Oomen, A. G. (2020). Possible Effects of Titanium Dioxide Particles on Human Liver, Intestinal Tissue, Spleen and Kidney after Oral Exposure. Nanotoxicology 14 (7), 985–1007. doi:10.1080/17435390.2020.1778809
Breitenbach, M., and Eckl, P. (2015). Introduction to Oxidative Stress in Biomedical and Biological Research. Biomolecules 5 (2), 1169–1177. doi:10.3390/biom5021169
Buonanno, M., de Toledo, S. M., Pain, D., and Azzam, E. I. (2011). Long-term Consequences of Radiation-Induced Bystander Effects Depend on Radiation Quality and Dose and Correlate with Oxidative Stress. Radiat. Res. 175 (4), 405–415. doi:10.1667/rr2461.1
Cadet, J., and Wagner, J. R. (2013). DNA Base Damage by Reactive Oxygen Species, Oxidizing Agents, and UV Radiation. Cold Spring Harb. Perspect. Biol. 5 (2), a012559. doi:10.1101/cshperspect.a012559
Califf, R. M. (2018). Biomarker Definitions and Their Applications. Exp. Biol. Med. (Maywood) 243 (3), 213–221. doi:10.1177/1535370217750088
Cecchini, R., and Cecchini, A. L. (2020). SARS-CoV-2 Infection Pathogenesis Is Related to Oxidative Stress as a Response to Aggression. Med. Hypotheses 143, 110102. doi:10.1016/j.mehy.2020.110102
Cheignon, C., Tomas, M., Bonnefont-Rousselot, D., Faller, P., Hureau, C., and Collin, F. (2018). Oxidative Stress and the Amyloid Beta Peptide in Alzheimer's Disease. Redox Biol. 14, 450–464. doi:10.1016/j.redox.2017.10.014
Chen, S., Wang, Y., Zhang, H., Chen, R., Lv, F., Li, Z., et al. (2019). The Antioxidant MitoQ Protects against CSE-Induced Endothelial Barrier Injury and Inflammation by Inhibiting ROS and Autophagy in Human Umbilical Vein Endothelial Cells. Int. J. Biol. Sci. 15 (7), 1440–1451. doi:10.7150/ijbs.30193
Clerbaux, L.-A., Amorim, M. J., Bal-Price, A., Batista Leite, S., Beronius, A., Bezemer, G. F. G., et al. (2022). COVID-19 through Adverse Outcome Pathways: Building Networks to Better Understand the Disease - 3rd CIAO AOP Design Workshop. Altex 39, 322–335. doi:10.14573/altex.2112161
Climent, M., Viggiani, G., Chen, Y.-W., Coulis, G., and Castaldi, A. (2020). MicroRNA and ROS Crosstalk in Cardiac and Pulmonary Diseases. Ijms 21 (12), 4370. doi:10.3390/ijms21124370
Clydesdale, G. J., Dandie, G. W., and Muller, H. K. (2001). Ultraviolet Light Induced Injury: Immunological and Inflammatory Effects. Immunol. Cell. Biol. 79 (6), 547–568. doi:10.1046/j.1440-1711.2001.01047.x
Conklin, K. A. (2004). Chemotherapy-Associated Oxidative Stress: Impact on Chemotherapeutic Effectiveness. Integr. Cancer Ther. 3 (4), 294–300. doi:10.1177/1534735404270335
Connors, J. M., and Levy, J. H. (2020). COVID-19 and its Implications for Thrombosis and Anticoagulation. Blood 135 (23), 2033–2040. doi:10.1182/blood.2020006000
Datla, S. R., and Griendling, K. K. (2010). Reactive Oxygen Species, NADPH Oxidases, and Hypertension. Hypertension 56 (3), 325–330. doi:10.1161/hypertensionaha.109.142422
De Logu, F., Souza Monteiro de Araujo, D., Ugolini, F., Iannone, L. F., Vannucchi, M., Portelli, F., et al. (2021). The TRPA1 Channel Amplifies the Oxidative Stress Signal in Melanoma. Cells 10 (11), 3131. doi:10.3390/cells10113131
Di Florio, D. N., Sin, J., Coronado, M. J., Atwal, P. S., and Fairweather, D. (2020). Sex Differences in Inflammation, Redox Biology, Mitochondria and Autoimmunity. Redox Biol. 31, 101482. doi:10.1016/j.redox.2020.101482
Dikalov, S. I., and Harrison, D. G. (2014). Methods for Detection of Mitochondrial and Cellular Reactive Oxygen Species. Antioxidants redox Signal. 20 (2), 372–382. doi:10.1089/ars.2012.4886
Đorđević, V., Stanković Đorđević, D., Kocić, B., Dinić, M., Sokolović, D., and Pešić Stanković, J. (2021). The Impact of Hepatitis C Virus Genotypes on Oxidative Stress Markers and Catalase Activity. Oxidative Med. Cell. Longev. 2021, 6676057. doi:10.1155/2021/6676057
Dröge, W. (2002). Free Radicals in the Physiological Control of Cell Function. Physiol. Rev. 82 (1), 47–95. doi:10.1152/physrev.00018.2001
Du, S., Miao, J., Lu, X., Shi, L., Sun, J., Xu, E., et al. (2019). NADPH Oxidase 4 Is Correlated with Gastric Cancer Progression and Predicts a Poor Prognosis. Am. J. Transl. Res. 11 (6), 3518–3530.
Flaherty, R. L., Owen, M., Fagan-Murphy, A., Intabli, H., Healy, D., Patel, A., et al. (2017). Glucocorticoids Induce Production of Reactive Oxygen Species/reactive Nitrogen Species and DNA Damage through an iNOS Mediated Pathway in Breast Cancer. Breast Cancer Res. 19 (1), 35–13. doi:10.1186/s13058-017-0823-8
Forrester, S. J., Booz, G. W., Sigmund, C. D., Coffman, T. M., Kawai, T., Rizzo, V., et al. (2018). Angiotensin II Signal Transduction: An Update on Mechanisms of Physiology and Pathophysiology. Physiol. Rev. 98 (3), 1627–1738. doi:10.1152/physrev.00038.2017
Frazziano, G., Al Ghouleh, I., Baust, J., Shiva, S., Champion, H. C., and Pagano, P. J. (2014). Nox-derived ROS Are Acutely Activated in Pressure Overload Pulmonary Hypertension: Indications for a Seminal Role for Mitochondrial Nox4. Am. J. Physiology-Heart Circulatory Physiology 306 (2), H197–H205. doi:10.1152/ajpheart.00977.2012
Freudenthal, B. D., Schaich, M. A., Smith, M. R., Flynn, T. S., and Freudenthal, B. D. (2017). Base Excision Repair of Oxidative DNA Damage from Mechanism to Disease. Front. Biosci. 22, 1493–1522. doi:10.2741/4555
Frisoni, P., Neri, M., D’Errico, S., Alfieri, L., Bonuccelli, D., Cingolani, M., et al. (2021). Cytokine Storm and Histopathological Findings in 60 Cases of COVID-19-Related Death: from Viral Load Research to Immunohistochemical Quantification of Major Players IL-1β, IL-6, IL-15 and TNF-α. Forensic Sci. Med. Pathol. 18, 4–19. doi:10.1007/s12024-021-00414-9
Fukai, T., and Ushio-Fukai, M. (2020). Cross-talk between NADPH Oxidase and Mitochondria: Role in ROS Signaling and Angiogenesis. Cells 9 (8), 1849. doi:10.3390/cells9081849
Fuloria, S., Subramaniyan, V., Karupiah, S., Kumari, U., Sathasivam, K., Meenakshi, D. U., et al. (2021). Comprehensive Review of Methodology to Detect Reactive Oxygen Species (ROS) in Mammalian Species and Establish its Relationship with Antioxidants and Cancer. Antioxidants 10 (1), 128. doi:10.3390/antiox10010128
Gebicki, J. M. (2016). Oxidative Stress, Free Radicals and Protein Peroxides. Archives Biochem. Biophysics 595, 33–39. doi:10.1016/j.abb.2015.10.021
Ghezzi, P. (2020). Environmental Risk Factors and Their Footprints In Vivo - A Proposal for the Classification of Oxidative Stress Biomarkers. Redox Biol. 34, 101442. doi:10.1016/j.redox.2020.101442
Gomes, T., Xie, L., Brede, D., Lind, O.-C., Solhaug, K. A., Salbu, B., et al. (2017). Sensitivity of the Green Algae Chlamydomonas Reinhardtii to Gamma Radiation: Photosynthetic Performance and ROS Formation. Aquat. Toxicol. 183, 1–10. doi:10.1016/j.aquatox.2016.12.001
Goodhead, D. T. (1988). Spatial and Temporal Distribution of Energy. Health Phys. 55 (2), 231–240. doi:10.1097/00004032-198808000-00015
Gornicka, A., Morris-Stiff, G., Thapaliya, S., Papouchado, B. G., Berk, M., and Feldstein, A. E. (2011). Transcriptional Profile of Genes Involved in Oxidative Stress and Antioxidant Defense in a Dietary Murine Model of Steatohepatitis. Antioxidants Redox Signal. 15 (2), 437–445. doi:10.1089/ars.2010.3815
Gu, H., Huang, T., Shen, Y., Liu, Y., Zhou, F., Jin, Y., et al. (2018). Reactive Oxygen Species-Mediated Tumor Microenvironment Transformation: The Mechanism of Radioresistant Gastric Cancer. Oxidative Med. Cell. Longev. 2018, 5801209. doi:10.1155/2018/5801209
Halappanavar, S., Ede, J. D., Mahapatra, I., Krug, H. F., Kuempel, E. D., Lynch, I., et al. (2021). A Methodology for Developing Key Events to Advance Nanomaterial-Relevant Adverse Outcome Pathways to Inform Risk Assessment. Nanotoxicology 15 (3), 289–310. doi:10.1080/17435390.2020.1851419
Halappanavar, S., van den Brule, S., Nymark, P., Gaté, L., Seidel, C., Valentino, S., et al. (2020). Adverse Outcome Pathways as a Tool for the Design of Testing Strategies to Support the Safety Assessment of Emerging Advanced Materials at the Nanoscale. Part Fibre Toxicol. 17 (1), 16. doi:10.1186/s12989-020-00344-4
Hanahan, D., and Weinberg, R. A. (2011). Hallmarks of Cancer: the Next Generation. Cell. 144 (5), 646–674. doi:10.1016/j.cell.2011.02.013
Hardie, W. D., Glasser, S. W., and Hagood, J. S. (2009). Emerging Concepts in the Pathogenesis of Lung Fibrosis. Am. J. pathology 175 (1), 3–16. doi:10.2353/ajpath.2009.081170
Hargreaves, M., and Spriet, L. L. (2020). Skeletal Muscle Energy Metabolism during Exercise. Nat. Metab. 2 (9), 817–828. doi:10.1038/s42255-020-0251-4
He, J., Liu, L., Tang, F., Zhou, Y., Liu, H., Lu, C., et al. (2021). Paradoxical Effects of DNA Tumor Virus Oncogenes on Epithelium-Derived Tumor Cell Fate during Tumor Progression and Chemotherapy Response. Sig Transduct. Target Ther. 6 (1), 408. doi:10.1038/s41392-021-00787-x
Helm, J. S., and Rudel, R. A. (2020). Adverse Outcome Pathways for Ionizing Radiation and Breast Cancer Involve Direct and Indirect DNA Damage, Oxidative Stress, Inflammation, Genomic Instability, and Interaction with Hormonal Regulation of the Breast. Arch. Toxicol. 94 (5), 1511–1549. doi:10.1007/s00204-020-02752-z
Henkler, F., Brinkmann, J., and Luch, A. (2010). The Role of Oxidative Stress in Carcinogenesis Induced by Metals and Xenobiotics. Cancers 2 (2), 376–396. doi:10.3390/cancers2020376
Ho, E., Karimi Galougahi, K., Liu, C.-C., Bhindi, R., and Figtree, G. A. (2013). Biological Markers of Oxidative Stress: Applications to Cardiovascular Research and Practice. Redox Biol. 1 (1), 483–491. doi:10.1016/j.redox.2013.07.006
Huang, X., He, D., Pan, Z., Luo, G., and Deng, J. (2021). Reactive-oxygen-species-scavenging Nanomaterials for Resolving Inflammation. Mater. Today Bio 11, 100124. doi:10.1016/j.mtbio.2021.100124
Huh, D., Matthews, B. D., Mammoto, A., Montoya-Zavala, M., Hsin, H. Y., and Ingber, D. E. (2010). Reconstituting Organ-Level Lung Functions on a Chip. Science 328 (5986), 1662–1668. doi:10.1126/science.1188302
Ishida, M., Ishida, T., Tashiro, S., Uchida, H., Sakai, C., Hironobe, N., et al. (2014). Smoking Cessation Reverses DNA Double-Strand Breaks in Human Mononuclear Cells. PLOS ONE 9 (8), e103993. doi:10.1371/journal.pone.0103993
Ivanov, A. V., Bartosch, B., and Isaguliants, M. G. (2017). Oxidative Stress in Infection and Consequent Disease. Oxidative Med. Cell. Longev. 2017, 3496043. doi:10.1155/2017/3496043
Jacobsen, N. R., Pojana, G., White, P., Møller, P., Cohn, C. A., Smith Korsholm, K., et al. (2008). Genotoxicity, Cytotoxicity, and Reactive Oxygen Species Induced by Single‐walled Carbon Nanotubes and C60fullerenes in the FE1‐MutaMouse Lung Epithelial Cells. Environ. Mol. Mutagen. 49, 476–487. doi:10.1002/em.20406
Jacobsen, N. R., Saber, A. T., White, P., Møller, P., Pojana, G., Vogel, U., et al. (2007). Increased Mutant Frequency by Carbon Black, but Not Quartz, in thelacZ andcII Transgenes of Mutamouse Lung Epithelial Cells. Environ. Mol. Mutagen. 48 (6), 451–461. doi:10.1002/em.20300
Jacobsen, N. R., White, P. A., Gingerich, J., Møller, P., Saber, A. T., Douglas, G. R., et al. (2011). Mutation Spectrum in FE1-MUTATMMouse Lung Epithelial Cells Exposed to Nanoparticulate Carbon Black. Environ. Mol. Mutagen. 52 (4), 331–337. doi:10.1002/em.20629
Jiang, D., Muschhammer, J., Qi, Y., Kügler, A., de Vries, J. C., Saffarzadeh, M., et al. (2016). Suppression of Neutrophil-Mediated Tissue Damage-A Novel Skill of Mesenchymal Stem Cells. STEM CELLS 34 (9), 2393–2406. doi:10.1002/stem.2417
Kaidashev, I., Shlykova, O., Izmailova, O., Torubara, O., Yushchenko, Y., Tyshkovska, T., et al. (2021). Host Gene Variability and SARS-CoV-2 Infection: A Review Article. Heliyon 7 (8), e07863. doi:10.1016/j.heliyon.2021.e07863
Kalyanaraman, B. (2021). Reactive Oxygen Species, Proinflammatory and Immunosuppressive Mediators Induced in COVID-19: Overlapping Biology with Cancer. RSC Chem. Biol. 2 (5), 1402–1414. doi:10.1039/d1cb00042j
Katerji, M., Filippova, M., and Duerksen-Hughes, P. (2019). Approaches and Methods to Measure Oxidative Stress in Clinical Samples: Research Applications in the Cancer Field. Oxidative Med. Cell. Longev. 2019, 1279250. doi:10.1155/2019/1279250
Katoh, Y., and Katoh, M. (2009). FGFR2-related Pathogenesis and FGFR2-Targeted Therapeutics (Review). Int. J. Mol. Med. 23 (3), 307–311. doi:10.3892/ijmm_00000132
Kay, J., Thadhani, E., Samson, L., and Engelward, B. (2019). Inflammation-induced DNA Damage, Mutations and Cancer. DNA Repair 83, 102673. doi:10.1016/j.dnarep.2019.102673
Khalil Alyahya, H., Subash-Babu, P., Mohammad Salamatullah, A., Hayat, K., Albader, N., Alkaltham, M. S., et al. (2021). Quantification of Chlorogenic Acid and Vanillin from Coffee Peel Extract and its Effect on α-Amylase Activity, Immunoregulation, Mitochondrial Oxidative Stress, and Tumor Suppressor Gene Expression Levels in H2O2-Induced Human Mesenchymal Stem Cells. Front. Pharmacol. 12 (2734), 760242. doi:10.3389/fphar.2021.760242
Kim, K. S., Lee, D., Song, C. G., and Kang, P. M. (2015). Reactive Oxygen Species-Activated Nanomaterials as Theranostic Agents. Nanomedicine 10 (17), 2709–2723. doi:10.2217/nnm.15.108
Klose, J., Li, L., Pahl, M., Bendt, F., Hübenthal, U., Jüngst, C., et al. (2022). Application of the Adverse Outcome Pathway Concept for Investigating Developmental Neurotoxicity Potential of Chinese Herbal Medicines by Using Human Neural Progenitor Cells In Vitro. Cell. Biol. Toxicol. doi:10.1007/s10565-022-09730-4
Kozak, J., Forma, A., Czeczelewski, M., Kozyra, P., Sitarz, E., Radzikowska-Büchner, E., et al. (2020). Inhibition or Reversal of the Epithelial-Mesenchymal Transition in Gastric Cancer: Pharmacological Approaches. Ijms 22 (1), 277. doi:10.3390/ijms22010277
Kuo, C.-Y., Yang, T.-H., Tsai, P.-F., and Yu, C.-H. (2021). Role of the Inflammatory Response of RAW 264.7 Cells in the Metastasis of Novel Cancer Stem-like Cells. Medicina 57 (8), 778. doi:10.3390/medicina57080778
Lee, D. Y., Kang, S., Lee, Y., Kim, J. Y., Yoo, D., Jung, W., et al. (2020). PEGylated Bilirubin-Coated Iron Oxide Nanoparticles as a Biosensor for Magnetic Relaxation Switching-Based ROS Detection in Whole Blood. Theranostics 10 (5), 1997–2007. doi:10.7150/thno.39662
Liguori, I., Russo, G., Curcio, F., Bulli, G., Aran, L., Della-Morte, D., et al. (2018). Oxidative Stress, Aging, and Diseases. Cia 13, 757–772. doi:10.2147/cia.S158513
Liou, G.-Y., and Storz, P. (2010). Reactive Oxygen Species in Cancer. Free Radic. Res. 44 (5), 479–496. doi:10.3109/10715761003667554
Lu, X., Zhu, T., Chen, C., and Liu, Y. (2014). Right or Left: The Role of Nanoparticles in Pulmonary Diseases. Ijms 15 (10), 17577–17600. doi:10.3390/ijms151017577
Lukaszewicz-Hussain, A. (2010). Role of Oxidative Stress in Organophosphate Insecticide Toxicity - Short Review. Pesticide Biochem. Physiology 98 (2), 145–150. doi:10.1016/j.pestbp.2010.07.006
Ma, E., Ingram, K. H., Milne, G. L., and Garvey, W. T. (2017). F2-Isoprostanes Reflect Oxidative Stress Correlated with Lean Mass and Bone Density but Not Insulin Resistance. J. Endocr. Soc. 1 (5), 436–448. doi:10.1210/js.2017-00006
Ma, S., Fu, X., Liu, L., Liu, Y., Feng, H., Jiang, H., et al. (2021). Iron-Dependent Autophagic Cell Death Induced by Radiation in MDA-MB-231 Breast Cancer Cells. Front. Cell. Dev. Biol. 9, 723801. doi:10.3389/fcell.2021.723801
Mackman, N., Antoniak, S., Wolberg, A. S., Kasthuri, R., and Key, N. S. (2020). Coagulation Abnormalities and Thrombosis in Patients Infected with SARS-CoV-2 and Other Pandemic Viruses. Atvb 40 (9), 2033–2044. doi:10.1161/ATVBAHA.120.314514
Meagher, E. A., and FitzGerald, G. A. (2000). Indices of Lipid Peroxidation In Vivo: Strengths and Limitations. Free Radic. Biol. Med. 28 (12), 1745–1750. doi:10.1016/s0891-5849(00)00232-x
Medithi, S., Jonnalagadda, P. R., and Jee, B. (2021). Predominant Role of Antioxidants in Ameliorating the Oxidative Stress Induced by Pesticides. Archives Environ. Occup. Health 76 (2), 61–74. doi:10.1080/19338244.2020.1750333
Milne, G. L., Yin, H., Hardy, K. D., Davies, S. S., and Roberts, L. J. (2011). Isoprostane Generation and Function. Chem. Rev. 111 (10), 5973–5996. doi:10.1021/cr200160h
Modrzynska, J., Berthing, T., Ravn-Haren, G., Jacobsen, N. R., Weydahl, I. K., Loeschner, K., et al. (2018). Primary Genotoxicity in the Liver Following Pulmonary Exposure to Carbon Black Nanoparticles in Mice. Part Fibre Toxicol. 15 (1), 2. doi:10.1186/s12989-017-0238-9
Nagata, Y., Kudo, M., Nagai, T., Watanabe, T., Kawasaki, M., Asakuma, Y., et al. (2013). Heat Shock Protein 27 Expression Is Inversely Correlated with Atrophic Gastritis and Intraepithelial Neoplasia. Dig. Dis. Sci. 58 (2), 381–388. doi:10.1007/s10620-012-2342-x
Nash, K. M., and Ahmed, S. (2015). Nanomedicine in the ROS-Mediated Pathophysiology: Applications and Clinical Advances. Nanomedicine Nanotechnol. Biol. Med. 11 (8), 2033–2040. doi:10.1016/j.nano.2015.07.003
Nguyen Dinh Cat, A., Montezano, A. C., Burger, D., and Touyz, R. M. (2013). Angiotensin II, NADPH Oxidase, and Redox Signaling in the Vasculature. Antioxidants redox Signal. 19 (10), 1110–1120. doi:10.1089/ars.2012.4641
Nishida, M., Tanabe, S., Maruyama, Y., Mangmool, S., Urayama, K., Nagamatsu, Y., et al. (2005). Gα12/13- and Reactive Oxygen Species-dependent Activation of C-Jun NH2-terminal Kinase and P38 Mitogen-Activated Protein Kinase by Angiotensin Receptor Stimulation in Rat Neonatal Cardiomyocytes. J. Biol. Chem. 280 (18), 18434–18441. doi:10.1074/jbc.M409710200
Nymark, P., Karlsson, H. L., Halappanavar, S., and Vogel, U. (2021). Adverse Outcome Pathway Development for Assessment of Lung Carcinogenicity by Nanoparticles. Front. Toxicol. 3 (21), 653386. doi:10.3389/ftox.2021.653386
Omidifar, N., Nili-Ahmadabadi, A., Nakhostin-Ansari, A., Lankarani, K. B., Moghadami, M., Mousavi, S. M., et al. (2021). The Modulatory Potential of Herbal Antioxidants against Oxidative Stress and Heavy Metal Pollution: Plants against Environmental Oxidative Stress. Environ. Sci. Pollut. Res. 28 (44), 61908–61918. doi:10.1007/s11356-021-16530-6
Onoue, S., Hosoi, K., Wakuri, S., Iwase, Y., Yamamoto, T., Matsuoka, N., et al. (2012). Establishment and Intra-/inter-laboratory Validation of a Standard Protocol of Reactive Oxygen Species Assay for Chemical Photosafety Evaluation. J. Appl. Toxicol. 33 (11), a–n. doi:10.1002/jat.2776
Organization (2020). Global Health Estimates 2020: Deaths by Cause, Age. Sex, by Country and by Region, 2000-2019. Available: https://www.who.int/data/gho/data/themes/mortality-and-global-health-estimates/ghe-leading-causes-of-death (Accessed December 16, 2021).
Ortiz-Prado, E., Dunn, J. F., Vasconez, J., Castillo, D., and Viscor, G. (2019). Partial Pressure of Oxygen in the Human Body: a General Review. Am. J. Blood Res. 9 (1), 1–14.
Parimon, T., Yao, C., Stripp, B. R., Noble, P. W., and Chen, P. (2020). Alveolar Epithelial Type II Cells as Drivers of Lung Fibrosis in Idiopathic Pulmonary Fibrosis. Ijms 21 (7), 2269. doi:10.3390/ijms21072269
Porter, N. A., Caldwell, S. E., and Mills, K. A. (1995). Mechanisms of Free Radical Oxidation of Unsaturated Lipids. Lipids 30 (4), 277–290. doi:10.1007/bf02536034
Pothen, L., and Balligand, J.-L. (2021). Legacy in Cardiovascular Risk Factors Control: From Theory to Future Therapeutic Strategies? Antioxidants 10 (11), 1849. doi:10.3390/antiox10111849
Powers, S. K., and Jackson, M. J. (2008). Exercise-Induced Oxidative Stress: Cellular Mechanisms and Impact on Muscle Force Production. Physiol. Rev. 88 (4), 1243–1276. doi:10.1152/physrev.00031.2007
Racanelli, A. C., Kikkers, S. A., Choi, A. M. K., and Cloonan, S. M. (2018). Autophagy and Inflammation in Chronic Respiratory Disease. Autophagy 14 (2), 221–232. doi:10.1080/15548627.2017.1389823
Renu, K., Chakraborty, R., Myakala, H., Koti, R., Famurewa, A. C., Madhyastha, H., et al. (2021). Molecular Mechanism of Heavy Metals (Lead, Chromium, Arsenic, Mercury, Nickel and Cadmium) - Induced Hepatotoxicity - A Review. Chemosphere 271, 129735. doi:10.1016/j.chemosphere.2021.129735
Rocha, J. L. M., de Oliveira, W. C. F., Noronha, N. C., dos Santos, N. C. D., Covas, D. T., Picanço-Castro, V., et al. (2020). Mesenchymal Stromal Cells in Viral Infections: Implications for COVID-19. Stem Cell. Rev Rep 17, 71–93. doi:10.1007/s12015-020-10032-7
Rojas-Sanchez, G., García-Miranda, A., Montes-Alvarado, J. B., Cotzomi-Ortega, I., Sarmiento-Salinas, F. L., Jimenez-Ignacio, E. E., et al. (2021). Chloroquine Induces ROS-Mediated Macrophage Migration Inhibitory Factor Secretion and Epithelial to Mesenchymal Transition in ER-Positive Breast Cancer Cell Lines. J. Mammary Gland. Biol. Neoplasia 26, 341–355. doi:10.1007/s10911-021-09503-5
Salbu, B., Teien, H. C., Lind, O. C., and Tollefsen, K. E. (2019). Why Is the Multiple Stressor Concept of Relevance to Radioecology? Int. J. Radiat. Biol. 95 (7), 1015–1024. doi:10.1080/09553002.2019.1605463
Saleh, J., Peyssonnaux, C., Singh, K. K., and Edeas, M. (2020). Mitochondria and Microbiota Dysfunction in COVID-19 Pathogenesis. Mitochondrion 54, 1–7. doi:10.1016/j.mito.2020.06.008
Sasaki, J. C., Allemang, A., Bryce, S. M., Custer, L., Dearfield, K. L., Dietz, Y., et al. (2020). Application of the Adverse Outcome Pathway Framework to Genotoxic Modes of Action. Environ. Mol. Mutagen 61 (1), 114–134. doi:10.1002/em.22339
Sauvaget, C., Lagarde, F., Nagano, J., Soda, M., Koyama, K., and Kodama, K. (2005). Lifestyle Factors, Radiation and Gastric Cancer in Atomic-Bomb Survivors (Japan). Cancer Causes Control 16 (7), 773–780. doi:10.1007/s10552-005-5385-x
Scandalios, J. G. (2005). Oxidative Stress: Molecular Perception and Transduction of Signals Triggering Antioxidant Gene Defenses. Braz J. Med. Biol. Res. 38 (7), 995–1014. doi:10.1590/s0100-879x2005000700003
Schaich, M. A., and Van Houten, B. (2021). Searching for DNA Damage: Insights from Single Molecule Analysis. Front. Mol. Biosci. 8, 772877. doi:10.3389/fmolb.2021.772877
Seebacher, N. A., Krchniakova, M., Stacy, A. E., Skoda, J., and Jansson, P. J. (2021). Tumour Microenvironment Stress Promotes the Development of Drug Resistance. Antioxidants 10 (11), 1801. doi:10.3390/antiox10111801
Seen, S., and Tong, L. (2018). Dry Eye Disease and Oxidative Stress. Acta Ophthalmol. 96 (4), e412–e420. doi:10.1111/aos.13526
Shenoy, S. (2020). Coronavirus (Covid-19) Sepsis: Revisiting Mitochondrial Dysfunction in Pathogenesis, Aging, Inflammation, and Mortality. Inflamm. Res. 69 (11), 1077–1085. doi:10.1007/s00011-020-01389-z
Shi, T., Denney, L., An, H., Ho, L. P., and Zheng, Y. (2021). Alveolar and Lung Interstitial Macrophages: Definitions, Functions, and Roles in Lung Fibrosis. J. Leukoc. Bio 110 (1), 107–114. doi:10.1002/jlb.3ru0720-418r
Sies, H., Berndt, C., and Jones, D. P. (2017). Oxidative Stress. Annu. Rev. Biochem. 86, 715–748. doi:10.1146/annurev-biochem-061516-045037
Sies, H. (2015). Oxidative Stress: a Concept in Redox Biology and Medicine. Redox Biol. 4, 180–183. doi:10.1016/j.redox.2015.01.002
Smith, K. A., Shepherd, J., Wakil, A., and Kilpatrick, E. S. (2011). A Comparison of Methods for the Measurement of 8-isoPGF2α: a Marker of Oxidative Stress. Ann. Clin. Biochem. 48 (Pt 2), 147–154. doi:10.1258/acb.2010.010151
Song, Y., Xie, L., Lee, Y., Brede, D. A., Lyne, F., Kassaye, Y., et al. (2020). Integrative Assessment of Low-Dose Gamma Radiation Effects on Daphnia Magna Reproduction: Toxicity Pathway Assembly and AOP Development. Sci. Total Environ. 705, 135912. doi:10.1016/j.scitotenv.2019.135912
Steffensen, I.-L., Dirven, H., Couderq, S., David, A., D’Cruz, S., Fernández, M., et al. (2020). Bisphenols and Oxidative Stress Biomarkers-Associations Found in Human Studies, Evaluation of Methods Used, and Strengths and Weaknesses of the Biomarkers. Ijerph 17 (10), 3609. doi:10.3390/ijerph17103609
Sung, H., Ferlay, J., Siegel, R. L., Laversanne, M., Soerjomataram, I., Jemal, A., et al. (2021). Global Cancer Statistics 2020: GLOBOCAN Estimates of Incidence and Mortality Worldwide for 36 Cancers in 185 Countries. CA A Cancer J. Clin. 71 (3), 209–249. doi:10.3322/caac.21660
Tanabe, S., Beaton, D., Chauhan, V., Choi, I., Danielsen, P. H., Delrue, N., et al. (2022). Report of the 1st and 2nd Mystery of Reactive Oxygen Species Conferences. Altex 39 (2), 336–338. doi:10.14573/altex.2203011
Tanabe, S., Quader, S., Cabral, H., and Ono, R. (2020). Interplay of EMT and CSC in Cancer and the Potential Therapeutic Strategies. Front. Pharmacol. 11, 904. doi:10.3389/fphar.2020.00904
Tanabe, S., Quader, S., Ono, R., Cabral, H., Aoyagi, K., Hirose, A., et al. (2021). Cell Cycle Regulation and DNA Damage Response Networks in Diffuse- and Intestinal-type Gastric Cancer. Cancers 13 (22), 5786. doi:10.3390/cancers13225786
Tharmalingam, S., Sreetharan, S., Kulesza, A. V., Boreham, D. R., and Tai, T. C. (2017). Low-Dose Ionizing Radiation Exposure, Oxidative Stress and Epigenetic Programing of Health and Disease. Radiat. Res. 188 (4.2), 525–538. doi:10.1667/rr14587.1
Tsai, C.-H., Shen, Y.-C., Chen, H.-W., Liu, K.-L., Chang, J.-W., Chen, P.-Y., et al. (2017). Docosahexaenoic Acid Increases the Expression of Oxidative Stress-Induced Growth Inhibitor 1 through the PI3K/Akt/Nrf2 Signaling Pathway in Breast Cancer Cells. Food Chem. Toxicol. 108 (Pt A), 276–288. doi:10.1016/j.fct.2017.08.010
Valko, M., Leibfritz, D., Moncol, J., Cronin, M. T. D., Mazur, M., and Telser, J. (2007). Free Radicals and Antioxidants in Normal Physiological Functions and Human Disease. Int. J. Biochem. Cell. Biol. 39 (1), 44–84. doi:10.1016/j.biocel.2006.07.001
Vargas-Mendoza, N., Angeles-Valencia, M., Morales-González, Á., Madrigal-Santillán, E. O., Morales-Martínez, M., Madrigal-Bujaidar, E., et al. (2021). Oxidative Stress, Mitochondrial Function and Adaptation to Exercise: New Perspectives in Nutrition. Life 11 (11), 1269. doi:10.3390/life11111269
Veith, C., Boots, A. W., Idris, M., van Schooten, F.-J., and van der Vliet, A. (2019). Redox Imbalance in Idiopathic Pulmonary Fibrosis: a Role for Oxidant Cross-Talk between NADPH Oxidase Enzymes and Mitochondria. Antioxidants redox Signal. 31 (14), 1092–1115. doi:10.1089/ars.2019.7742
Wang, Y., Xu, M., Ke, Z.-j., and Luo, J. (2017). Cellular and Molecular Mechanisms Underlying Alcohol-Induced Aggressiveness of Breast Cancer. Pharmacol. Res. 115, 299–308. doi:10.1016/j.phrs.2016.12.005
Xie, L., Solhaug, K. A., Song, Y., Brede, D. A., Lind, O. C., Salbu, B., et al. (2019). Modes of Action and Adverse Effects of Gamma Radiation in an Aquatic Macrophyte Lemna Minor. Sci. Total Environ. 680, 23–34. doi:10.1016/j.scitotenv.2019.05.016
Yamamoto, M., Sanomachi, T., Suzuki, S., Togashi, K., Sugai, A., Seino, S., et al. (2021). Gemcitabine Radiosensitization Primes Irradiated Malignant Meningioma Cells for Senolytic Elimination by Navitoclax. Neurooncol Adv. 3 (1), vdab148. doi:10.1093/noajnl/vdab148
Yang, M., and Chitambar, C. R. (2008). Role of Oxidative Stress in the Induction of metallothionein-2A and Heme Oxygenase-1 Gene Expression by the Antineoplastic Agent Gallium Nitrate in Human Lymphoma Cells. Free Radic. Biol. Med. 45 (6), 763–772. doi:10.1016/j.freeradbiomed.2008.05.031
Yao, Y., Zang, Y., Qu, J., Tang, M., and Zhang, T. (2019). The Toxicity of Metallic Nanoparticles on Liver: The Subcellular Damages, Mechanisms, and Outcomes. Ijn 14, 8787–8804. doi:10.2147/ijn.S212907
Yi, X., Long, L., Yang, C., Lu, Y., and Cheng, M. (2014). Maotai Ameliorates Diethylnitrosamine-Initiated Hepatocellular Carcinoma Formation in Mice. PLoS One 9 (4), e93599. doi:10.1371/journal.pone.0093599
Yusefi, A. R., Bagheri Lankarani, K., Bastani, P., Radinmanesh, M., and Kavosi, Z. (2018). Risk Factors for Gastric Cancer: A Systematic Review. Asian Pac J. Cancer Prev. 19 (3), 591–603. doi:10.22034/apjcp.2018.19.3.591
Zdrojewicz, Z., Szlagor, A., Wielogórska, M., Nowakowska, D., and Nowakowski, J. (2016). Influence of Ionizing Radiation on Human Body. fmpcr 2 (2), 174–179. doi:10.5114/fmpcr/43945
Zhang, Y., Dai, M., and Yuan, Z. (2018). Methods for the Detection of Reactive Oxygen Species. Anal. Methods 10 (38), 4625–4638. doi:10.1039/C8AY01339J
Keywords: adverse outcome pathway (AOP), oxidative stress, reactive nitrogen species (RNS), disease, reactive oxygen species (ROS)
Citation: Tanabe S, O’Brien J, Tollefsen KE, Kim Y, Chauhan V, Yauk C, Huliganga E, Rudel RA, Kay JE, Helm JS, Beaton D, Filipovska J, Sovadinova I, Garcia-Reyero N, Mally A, Poulsen SS, Delrue N, Fritsche E, Luettich K, La Rocca C, Yepiskoposyan H, Klose J, Danielsen PH, Esterhuizen M, Jacobsen NR, Vogel U, Gant TW, Choi I and FitzGerald R (2022) Reactive Oxygen Species in the Adverse Outcome Pathway Framework: Toward Creation of Harmonized Consensus Key Events. Front.Toxicol. 4:887135. doi: 10.3389/ftox.2022.887135
Received: 16 March 2022; Accepted: 13 June 2022;
Published: 06 July 2022.
Edited by:
Stephanie Melching-Kollmuss, BASF, GermanyReviewed by:
Maciej Stepnik, QSAR Lab Ltd., PolandMichael McMahon, Penman Consulting, United Kingdom
Copyright © 2022 Tanabe, O’Brien, Tollefsen, Kim, Chauhan, Yauk, Huliganga, Rudel, Kay, Helm, Beaton, Filipovska, Sovadinova, Garcia-Reyero, Mally, Poulsen, Delrue, Fritsche, Luettich, La Rocca, Yepiskoposyan, Klose, Danielsen, Esterhuizen, Jacobsen, Vogel, Gant, Choi and FitzGerald. This is an open-access article distributed under the terms of the Creative Commons Attribution License (CC BY). The use, distribution or reproduction in other forums is permitted, provided the original author(s) and the copyright owner(s) are credited and that the original publication in this journal is cited, in accordance with accepted academic practice. No use, distribution or reproduction is permitted which does not comply with these terms.
*Correspondence: Shihori Tanabe, stanabe@nihs.go.jp